Revolutionizing barium ion detection for neutrino research
In the quest to unravel the mysteries of the universe, scientists are turning to tiny glowing molecules and powerful microscopes to detect elusive particles. Two groundbreaking studies, one published in ACS Sensors in 2025 and another in Nature Communications in 2024, showcase innovative approaches to detecting barium ions in high-pressure xenon gas. These advancements are pivotal for experiments searching for neutrinoless double beta decay, a rare process that could reveal whether neutrinos are their own antiparticles, potentially reshaping our understanding of particle physics and the matter-antimatter imbalance in the universe. Let’s explore how these glowing sensors and microscopes work, their significance, and what they mean for the future.
The glowing sensor: A chemical detective for barium
The ACS Sensors study introduces 1 a remarkable molecule based on iridium, designed to act like a chemical detective that lights up when it finds a barium ion. Barium ions, positively charged barium atoms, are key to detecting neutrinoless double beta decay, where a xenon-136 atom decays into a barium ion and two electrons. Spotting this ion alongside the electrons would provide strong evidence of the decay, a process that could confirm neutrinos as Majorana particles—particles that are their own antiparticles.
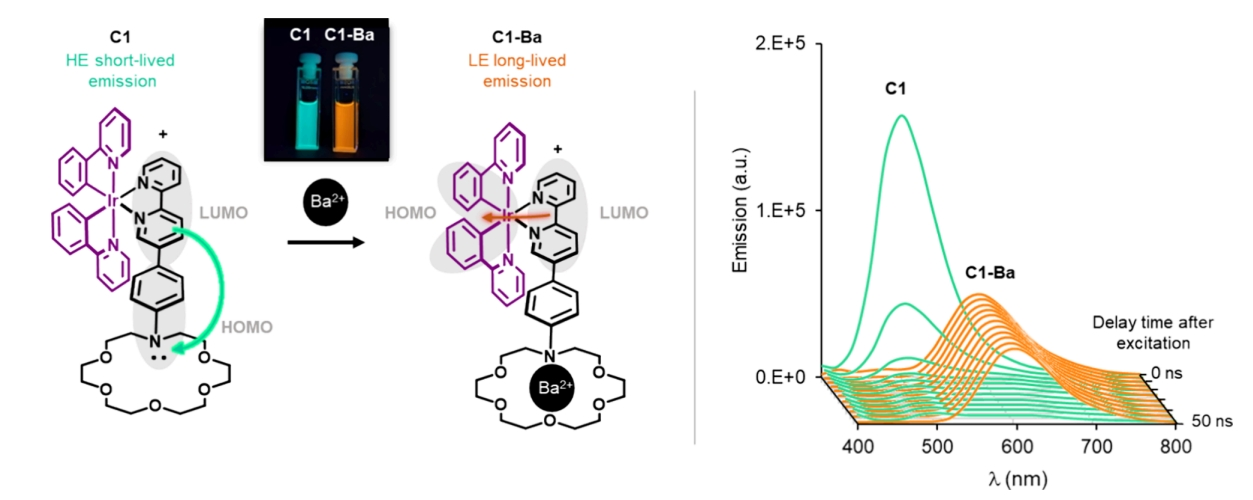
This iridium-based molecule is a “turn-on” sensor, meaning it changes its light output dramatically when it binds to a barium ion. When alone, it emits a quick burst of greenish fluorescence, lasting just a few nanoseconds. But when it captures a barium ion, it switches to a longer-lasting orange-red phosphorescence, glowing for over a microsecond. This shift is like a traffic light changing from green to red, making it easy to distinguish whether the molecule has found its target. The molecule’s design includes a crown ether, a ring-shaped structure that acts like a trap for barium ions, ensuring high sensitivity. The researchers tested it in acetonitrile, a solvent, using ultraviolet light to excite the molecule and measure its glow. They found that the free molecule’s fluorescence peaks at a wavelength producing green light, while the barium-bound molecule’s phosphorescence peaks at a longer wavelength, creating the orange-red glow.
This sensor’s ability to detect single barium ions is crucial for experiments like those conducted by the NEXT Collaboration, where even one ion could signal a rare decay event. However, the sensor isn’t perfect—it can also bind to other ions like calcium or strontium, which could cause false signals in complex environments. Fortunately, in the controlled setting of a xenon gas detector, where barium ions are the primary target, this cross-reactivity is less of a concern.
The high-pressure microscope: Seeing single barium ions
Complementing the glowing sensor, the Nature Communications study unveils 2 a high-pressure fluorescence microscope capable of imaging individual barium ions in 10 bar of xenon gas. This microscope uses single-molecule fluorescence imaging (SMFI), a Nobel Prize-winning technique that allows scientists to see molecules one at a time. The setup is designed to work at the gas-solid interface, where barium ions are captured by a chemosensor called Ion Potassium Green (IPG-1), coated onto a glass slide.
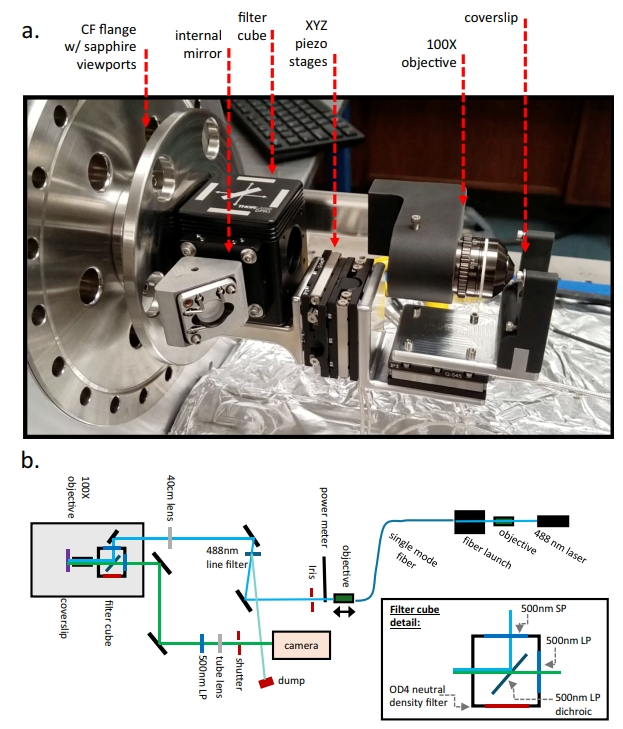
The microscope employs a 510 nm laser to excite the IPG-1 molecules, which glow brightly when bound to barium ions. The system is diffraction-limited, meaning it achieves the highest possible resolution for visible light, resolving features as small as a few hundred nanometers. It scans a 1 x 1 cm² area, using a customized 100X objective lens to focus light in the high-pressure environment. The emitted fluorescence is captured by an electron-multiplying CCD camera, which detects the faint glow of single molecules. To ensure clear images, the system uses filters to remove background noise from glass fluorescence and thermal camera noise, and an autofocus algorithm keeps the image sharp across the scan area.
The results are striking. When barium ions are present, the microscope reveals bright spots of fluorescence, each corresponding to a single ion bound to an IPG-1 molecule. These spots show characteristic behaviors like photobleaching (where the molecule stops glowing after prolonged light exposure) and photoblinking (temporary flickering), confirming they come from single molecules. The study found that in xenon gas, the photobleaching lifetime is 70.6 seconds, much longer than the 15.9 seconds in air, suggesting that oxygen in air accelerates the bleaching process. This longer lifetime in xenon is a boon for experiments needing prolonged observation.
Why it matters: Chasing neutrinoless double beta decay
Both studies are driven by the same goal: detecting neutrinoless double beta decay in xenon-136, which decays to barium-136 plus two electrons. This process, if observed, would violate lepton number conservation, confirm neutrinos as Majorana particles, and provide clues about why the universe has more matter than antimatter. Current experiments, like those using the KamLAND-Zen detector, have set a lifetime limit of 2.3 x 10²⁶ years for this decay, but detecting it requires ultra-low background noise and high sensitivity.
The iridium-based sensor and the high-pressure microscope tackle this challenge by enabling “barium tagging”—identifying the barium ion produced in the decay. By combining the sensor’s ability to glow selectively with the microscope’s power to image single ions, scientists can potentially eliminate background noise from other particles, making a detection nearly unmistakable. This could allow experiments to scale up to ton-sized detectors, increasing the chances of observing this rare event.
Challenges and future directions
Despite their promise, both technologies face hurdles. The iridium sensor’s cross-reactivity with other ions needs refinement for broader applications, though it’s less critical in xenon detectors. The microscope, while impressive, generates massive data volumes (15 GB for a single raster scan), requiring efficient data reduction algorithms for real-time use. Additionally, the current spin-coated molecular layer is sparse; future detectors will need densely packed fluorophores to capture every barium ion. The NEXT Collaboration is already working on these issues, exploring ion transport devices like radiofrequency carpets to guide ions to the sensor and testing densely packed molecular layers.
Beyond neutrino research, these technologies have wider potential. The iridium sensor’s fluorescence-phosphorescence switch could be adapted for medical imaging, detecting ions in biological systems, or environmental monitoring, tracking pollutants in water. The high-pressure microscope could study catalysts or sensors at the gas-solid interface, opening new avenues in chemistry and materials science.
Together, the glowing iridium sensor and the high-pressure fluorescence microscope represent a leap forward in barium ion detection. By lighting up and imaging single ions in xenon gas, these tools bring scientists closer to detecting neutrinoless double beta decay, a discovery that could redefine particle physics. They also showcase the power of combining chemistry and physics to solve complex problems, proving that even the faintest glow can illuminate the universe’s deepest secrets.
Author: César Tomé López is a science writer and the editor of Mapping Ignorance
Disclaimer: Parts of this article may have been copied verbatim or almost verbatim from the referenced research paper/s.
References
- Ane I. Aranburu, Mikel Elorza, Pablo R.G. Valle, Ariadna Pazos, Alexey Brodolin, Pablo Herrero-Gómez, J. Eduardo Barcelon, Gabriel Molina-Terriza, Francesc Monrabal, Celia Rogero, Fernando P. Cossío, Juan José Gómez-Cadenas, Claire Tonnelé, Zoraida Freixa, and the NEXT Collaboration (2025) Iridium-Based Time-Resolved Luminescent Sensor for Ba2+ Detection ACS Sensors doi: 10.1021/acssensors.4c01892 ↩
- Byrnes, N.K., Dey, E., Foss, F.W. et al. (2024) Fluorescence imaging of individual ions and molecules in pressurized noble gases for barium tagging in 136Xe. Nat Commun doi: 10.1038/s41467-024-54872-0 ↩