What is photodynamic therapy? Insights from computational chemistry
Author: Martina De Vetta is a Ph.D. student (ITN-EJD-TCCM) at Universidad Autónoma de Madrid
The increasing number of types and subtypes of cancer and their incidence 1 have triggered research to develop more selective alternative and less painful treatments compared to conventional therapies. 2
Photodynamic therapy (PDT) is an innovative treatment against certain types of cancer and other pre-cancerous lesions, which conventionally exploits the interaction between light, molecular oxygen and a photosensitive drug (PS).
![Figure 1. a) Electronic structure of the oxygen in its ground (3Σg-) and reactive excited state (1Δg). [3] b) Scheme of the key step in the PDT mechanism: an excited triplet state of the PS (3PSᶦ) transfers its energy to environmental oxygen.](https://mappingignorance.org/app/uploads/2016/11/Figure-1-640x323.png)
PDT works through the production of very reactive oxygen species, which can oxidize biological molecules compromising the normal functions of the targeted cells. The most toxic among these products is the lowest-lying singlet excited state of the O2 molecule (1Δg). As shown by Figure 1a, this excitation implies a different arrangement of the electrons in the π* orbitals compared to the ground state of the molecule: the “orientation” (spin) of the two electrons in these orbitals is opposite. Generation of singlet oxygen requires therefore a change in the multiplicity of the O2 molecule, which has a triplet multiplicity in its fundamental state (3Σg–). Electronic transitions, as the one that undergoes GS oxygen to generate 1O2 (1Δg), that imply a change in the molecule spin, are forbidden according to one of the selection rules that assess the probability for a certain excitation to occur.
In PDT the production of these reactive 1O2 species is achieved through the interaction between a photosensitive drug (PS) and oxygen in its fundamental state, as shown by Figure 1b. The PS should carry enough energy to promote the otherwise forbidden excitation from 3O2 to 1O2. Thus, the PS will be electronically excited.
Since the fundamental state of the PS has singlet multiplicity, the most probable transitions of the photosensitive drug will involve singlet excited states (1PSᶦ). The electronic states populated upon light absorption are called bright or spectroscopic states.
In PDT, light sources are limited by additional requirements that concern medical optimal conditions. The PS should have, in fact, specific absorption in the so-called “therapeutic window”, a property that has to be tuned in the design of efficient PSs. Irradiation in the range of 600-800 nm 3 minimizes light scattering favours light absorption of the tissues and thus allow the use of minimal doses of PS. 4
Referring again to Figure 1b, 1O2 is produced via energy transfer from a triplet excited state of the PS (3PSᶦ) to environmental ground state triplet oxygen 3O2, recovering the PS in its original ground state.5 Triplet excited states (3PSᶦ) have, in fact, longer lifetimes compared to their singlet counterparts allowing a more efficient interaction with molecular oxygen, leading to the formation of PS-O2 complexes. Afterwards, the dissociation of this complex yields the oxygen reactive species. 6
The success of PDT relies in its potential high selectivity: the PS is expected to selectively accumulate in the tumour tissue, which is subsequently irradiated with light.
So, the key step of PDT mechanism would be to bring the PS from its ground state (1PS) to its triplet excited state (3PSᶦ): this transition, again forbidden by the selection rules, is achieved through a cascade of deactivation processes initiated from the spectroscopic state(s) of the photosensitizer (1PSᶦ).
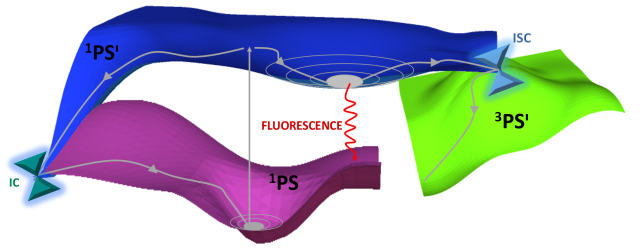
The deactivation processes in the PS can be explained in more detail with the help of Figure 2. Upon irradiation, the PS will populate one or more singlet excited states (1PSᶦ). To reach the triplet excited state, which is the reactive electronic state of the PS in photodynamical processes, a singlet-triplet intersystem crossing (ISC), preceded in some cases by internal conversion (IC) processes, has to take place. Both ISC and IC are non-radiative processes. ISC involves two states of different multiplicity and is therefore less probable than IC, which happens between excited states of the same multiplicity. Finally, a strong competing process to the essential ISC step for PDT, is fluorescence in which the molecule returns to its fundamental state in a radiative way. [6-7]
One of the challenges in PDT is to design photosensitive compounds that present accessible triplet excited states with lifetimes that persist long enough to let the PS-O2 complex to be formed and yield the active oxygen species.
For this purpose, careful examination of the topology of the potential energy surfaces of possible PSs, by means of computational approaches, can help identifying the possible deactivation pathways activated upon light absorption. Based on energetic and geometric criteria it is possible to establish the most probable deactivation funnels,7 although for deciphering the current relaxation mechanism it is necessary to resort to dynamical analysis of the problem. 8 Moreover, this time resolved approach not only allows identifying the chromophores showing efficient S-T ISCs but also is able to estimate the lifetime of the triplet reactive state, fundamental in photodynamical processes.
In summary, the predictive power of computational chemistry is extremely helpful for the design of new PSs with improved properties.
To take home: Computational chemistry can be a very helpful tool to assist in the design of more efficient photosensitizers. In silico approaches allow the screening of the optical and photophysical properties of a large number of photosensitizers at a relative low cost, and might contribute identifying the effect of the substitution in the absorption spectrum of chromophores. |
.
References
- American Cancer Society. Cancer Facts & Figures 2016. Cancer Facts Fig. 2016 1–9 (2016). ↩
- Soria, J. C. Annals of oncology: An editorial perspective. Ann. Oncol. 25, 5–6 (2014). ↩
- Zhu, T. C. & Finlay, J. C. The role of photodynamic therapy (PDT) physics. Med. Phys. 35, 3127 (2008). ↩
- DeRosa, M. C. & Crutchley, R. J. Photosensitized singlet oxygen and its applications. Coord. Chem. Rev. 233–234, 351–371 (2002). ↩
- Kamkaew, A. et al. BODIPY dyes in photodynamic therapy. Chem. Soc. Rev. 42, 77–88 (2013). ↩
- Awuah, S. G. & You, Y. Boron dipyrromethene (BODIPY)-based photosensitizers for photodynamic therapy. RSC Adv. 2, 11169 (2012). ↩
- Garavelli, M. Computational Organic Photochemistry: Strategy, Achievements and Perspectives. Theor. Chem. Acc. 116, 87–105 (2006). ↩
- González, L., Escudero, D. & Serrano-Andrés, L. Progress and challenges in the calculation of electronic excited states. ChemPhysChem 13, 28–51 (2012). ↩