Methane and the determination of the Majorana nature of neutrinos
Experiments performed in 1909 by Geiger and Marsden, also called Rutherford gold foil experiment because Rutherford was their supervisor, led to the discovery of nuclear structure in the atom: the nucleus of the atom is its central core and contains most of its mass and the nucleus is positively charged. Further research during the next decades showed that the nucleus consists of particles called nucleons. Nucleons can be either neutrons, with no electric charge, or protons with a positive electric charge. The total number of nucleons is usually called A, and the number of protons is called Z. The simplest nucleus is that of hydrogen with just one nucleon, a proton.
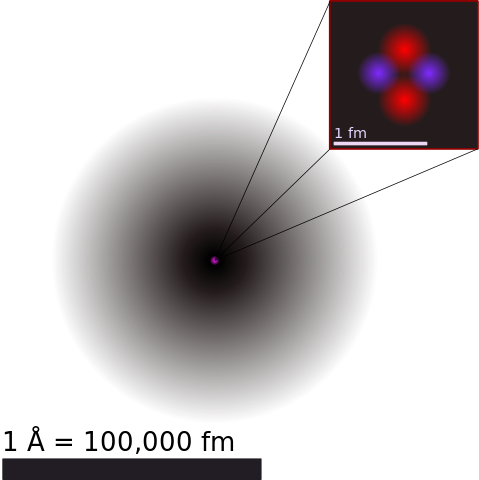
Thus, in a standard stable nucleus we have some neutrons and protons (summing up A nucleons), and its electric charge is equal to the number of protons, Z.
But not all nuclei are stable. Some are radioactive, meaning that some kind of decomposition takes place with the net result that there is a change in the nature of the nucleus and that some radiation, in many cases in the form of particles, is emitted.
One of this radioactive processes is called beta decay. There are three varieties of beta decay but we are interested in what follows only in the so-called negative beta decay, because one of particles issued is an electron. In this case, a neutron is transformed into a proton (A is constant but Z has increased in one, yielding a different chemical element) and an electron. But it is not that easy. When you try to balance the energy before and after the transformation you find there is a lack of energy in the products. Because the conservation of energy is the closest to a dogma there is in physics, Wolfgang Pauli postulated in 1930 that there should exist a tiny neutral particle that would account for the missing energy. He called this particle the neutrino and its existence was confirmed experimentally for good in 1956.
Now, you can ask about the properties of these neutrinos. Some of them have answers already: there are three classes of them and neutrinos can change between them, they have no charge, they move very close to the speed of light and have a non-zero mass (we do not know exactly how much, though). But there is another one which is much more difficult to answer and with important implications.
Particles usually have antiparticles. Antiparticles have the same mass as the particle it takes the name from but the opposite values of a different property. In the case of the electron, its antiparticle, the antielectron or positron, has the same mass but a positive charge. In the case of the neutron, the antineutron has the same mass but an opposite-sign magnetic moment relative to its spin. What about neutrinos? We do not know yet. There could be an antineutrino with the same mass but different chirality the neutrino has. If this is the case, in negative beta decay the particle emitted together with the electron would be an antineutrino.
If neutrinos are so-called Dirac particles then they are different from their antiparticles. But there is also the possibility that the neutrino is its own antiparticle. And when particle and antiparticle meet they annihilate. So, if neutrinos are their own antiparticle, they would be so-called Majorana particles, in a negative beta decay process, for every pair of atoms we may have two antineutrino-neutrino that would annihilate. In this annihilation more energy is produced, and this peak of energy should be detectable in the kinetic energy distribution of the electrons emitted.
Concluding that the neutrino is a Majorana particle would be really a turning point in our understanding of the universe. It would mean the non-conservation of a magnitude called the leptonic number, one of the key characteristics in a fermion, meaning that there would be an explanation to the matter-anti matter asymmetry: the creation of slightly different amounts of matter and antimatter in the Big Bang that, once annihilation finished, left a small amount of standard matter that we call our observable universe.
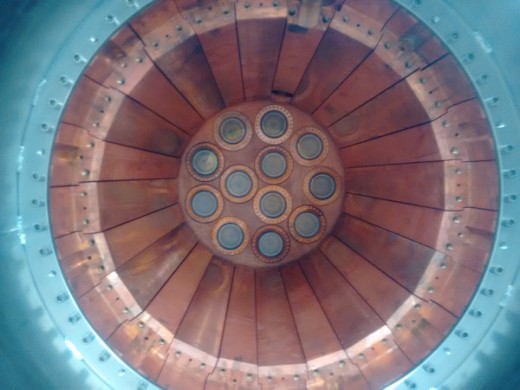
The NEXT experiment is trying to determine whether neutrinos are Majorana particles or not (you can read about it here and here). Basically NEXT uses the electroluminescence ampli fication – which provides a large yield of photons as a signal – of the primary ionisation signal provoked by emitted electrons in a volume of xenon-136-atom pressurized gas. The presence of molecular impurities in the noble gas was supposed to dramatically reduce the electroluminiscence yield. If an electron has a significant probability of colliding with a molecular impurity before it obtains from the electric field enough energy to excite a noble gas atom, it may lose part of its energy without producing electroluminiscence photons.
But why should NEXT tolerate any of these impurities in the xenon gas? The short answer is to make it better at what it is supposed to do. The longer answer is that, because the energy loss in elastic collisions with xenon atoms, there is a large electron diffusion, making the topological signature of the ionisation trail in the gas more difficult to measure; by adding a molecular gas, like CO2, CH4 or CF4, to pure xenon, new molecular degrees of freedom from vibrational and rotational states are made available for electron energy transfer in inelastic collisions, reducing considerably electron diffusion.
Recent experimental studies with Xe-CO2 and Xe-CH4 mixtures, for different concentrations of the additives, have shown that the reduction is not as drastic as previously believed. Hence, a campaign designed to systematically add several molecular gases to xenon, at minute concentration levels, has started within the scope of the NEXT experiment 1, with the participation of Ikerbasque Research Professor J.J. Gómez-Cadenas (DIPC), Ikerbasque Research Associate Paola Ferrario (DIPC) and Francesc Monrabal (DIPC & University of Texas, Arlington). The aim is to find a suitable mixture able to reduce diffusion and improve the topological discrimination of the events, without compromising significantly the performance of the detector in terms of electroluminescence yield and energy resolution.
The results show CO2 and CH4 show potential as molecular additives in a large xenon detector. While CO2 has some operational constraints, making it difficult to be used in a large one, CH4 shows the best performance and stability as molecular additive to be used in the NEXT-100 TPC, with an extrapolated energy resolution only slightly worse than the one obtained for pure xenon. Hence, if methane (or carbon dioxide) is used as an additive the detector could work very close to the diffusion limit imposed by the differences in temperature.
Author: César Tomé López is a science writer and the editor of Mapping Ignorance
References
- The NEXT Collaboration (2019) Electroluminescence TPCs at the thermal di usion limit Journal of High Energy Physics doi: 10.1007/JHEP01(2019)027 ↩