How to detect the daughter atom of a neutrinoless double beta decay
A new fluorescent bicolour indicator, an organic molecule, could help detect the daughter atom of a neutrinoless double beta decay. If this is achieved, there would be an explanation to the matter-anti matter asymmetry in the universe.
Experiments performed in 1909 by Geiger and Marsden, also called Rutherford gold foil experiment because Rutherford was their supervisor, led to the discovery of nuclear structure in the atom: the nucleus of the atom is its central core and contains most of its mass and the nucleus is positively charged. Further research during the next decades showed that the nucleus consists of particles called nucleons. Nucleons can be either neutrons, with no electric charge, or protons with a positive electric charge. The total number of nucleons is usually called A, and the number of protons is called Z. The simplest nucleus is that of hydrogen with just one nucleon, a proton.
Thus, in a standard stable nucleus we have some neutrons and protons (summing up A nucleons), and its electric charge is equal to the number of protons, Z.
But not all nuclei are stable. Some are radioactive, meaning that some kind of decomposition takes place with the net result that there is a change in the nature of the nucleus and that some radiation, in many cases in the form of particles, is emitted.
One of this radioactive processes is called beta decay. There are three varieties of beta decay but we are interested in what follows only in the so-called negative double beta decay, because one of particles issued is an electron. In this case, two neutrons are transformed into two protons: A is constant but Z has increased in two, yielding a different chemical element, so-called daughter element and an electron. But it is not that easy. When you try to balance the energy before and after the transformation you find there is a lack of energy in the products. Because the conservation of energy is the closest to a dogma there is in physics, Wolfgang Pauli postulated in 1930 that there should exist a tiny neutral particle that would account for the missing energy. He called this particle the neutrino and its existence was confirmed experimentally for good in 1956.
Now, you can ask about the properties of these neutrinos. Some of them have answers already. We now know that there are three classes of neutrinos, and a neutrino can change among them. We also know that neutrinos have no charge, that they move very close to the speed of light and that they have a non-zero mass, only that we do not know exactly how much, though. But there is another question which is much more difficult to answer and with important implications.
Are neutrinos Majorana particles?
Particles usually have antiparticles. Antiparticles have the same mass as the particle it takes the name from but the opposite values of a different property. In the case of the electron, its antiparticle, the antielectron or positron, has the same mass but a positive charge. In the case of the neutron, the antineutron has the same mass but an opposite-sign magnetic moment relative to its spin. What about neutrinos? We simply do not know yet. There could be an antineutrino with the same mass but different chirality the neutrino has. If this is the case, in negative beta decay the particle emitted together with the electron would be an antineutrino.
If neutrinos are like ‘normal’ particles, the so-called Dirac particles, then they differ from their antiparticles. But there is also the possibility that the neutrino is its own antiparticle, and then they would be so-called Majorana particles.
But, wait a minute. We know that when particle and antiparticle meet they annihilate. So, if neutrinos are their own antiparticle, in a negative beta decay process, for every pair of atoms we may have two antineutrino-neutrino that would annihilate, and the overall process would be neutrinoless. In this annihilation more energy would be produced, and this peak of energy should be detectable in the kinetic energy distribution of the electrons emitted.
Concluding that the neutrino is a Majorana particle would be really a turning point in our understanding of the universe. It would mean the non-conservation of a magnitude called the leptonic number, one of the key characteristics in a fermion, meaning that there would be an explanation to the matter-anti matter asymmetry: the creation of slightly different amounts of matter and antimatter in the Big Bang that, once annihilation finished, left a small amount of standard matter that we call our observable universe. Nobel-Prize-worth stuff.
Detecting a neutrinoless double beta decay
Hence, observation of the neutrinoless double beta decay is the only practical way to establish that neutrinos are their own antiparticles. But, because of the small masses of neutrinos, the lifetime of neutrinoless double beta decay is expected to be at least ten orders of magnitude greater than the typical lifetimes of natural radioactive chains, which can mimic the experimental signature of neutrinoless double beta decay. Therefore, the most robust identification of neutrinoless double beta decay requires the definition of a signature signal—such as the observation of the daughter atom in the decay—that cannot be generated by radioactive backgrounds, as well as excellent energy resolution.
All double beta experiments are built with ultrapure materials, operate in underground laboratories (to mitigate the impact of cosmic rays) and are protected by massive, ultrapure shields. These strategies reduce the ambient background by many orders of magnitude, but putative neutrinoless double beta decay events must still be extracted against tens of millions of spurious interactions.
Double beta decay experiments have been searching for neutrinoless events in several isotopes for more than half a century, without finding clear evidence of a signal so far. The current best lower limit on the lifetime of the neutrinoless double beta decay processes has been obtained for an isotope of xenon, 136Xe. In this case the daughter atom is an isotope of barium, 136Ba2+, the whole process being 136Xe → 136Ba2++ 2e– + 2 neutrinos. It seems only logical that the implementation of a robust Ba2+ detection technique would lead to a positive identification of a neutrinoless double beta decay candidate. This was proposed back in 1991 and has been extensively investigated for the past two decades.
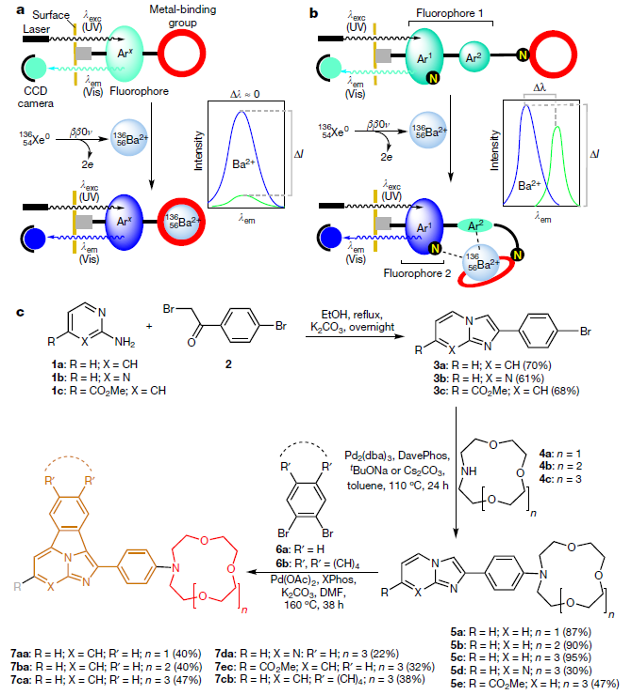
Now, a team of researchers proposes 1 a fluorescent bicolour indicator as the core of a sensor that can detect single Ba2+ ions in a high-pressure xenon gas detector.
A molecule with a response to optical stimulation that changes when it forms a supramolecular complex with a specific ion is a fluorescent indicator, and ions non-covalently bound in this way to molecules are generally referred to as being chelated.
The new indicator is designed (see figure) to bind strongly to Ba2+ and to shine very brightly when complexed with it. The emission spectrum of the chelated indicator is considerably blue-shifted with respect to the unchelated species, allowing an additional discrimination of almost two orders of magnitude.
In a sensor made of a monolayer of such indicators, Ba2+ would be captured by one of the molecules. The presence of such a single Ba2+-coordinated indicator would be revealed by its response to repeated interrogation with a laser system, enabling the development of a sensor able to detect single Ba2+ ions in high-pressure xenon gas detectors for barium-tagging experiments.
The proof of concept was done experimentally by sublimating barium perchlorate (Ba(ClO4)2) on fluorescent bicolour indicator molecules deposited on a silica pellet and interrogating the indicators using two-photon absorption microscopy. This is the first time that the formation of a Ba2+ supramolecular complex in a dry medium is demonstrated.
Author: César Tomé López is a science writer and the editor of Mapping Ignorance
Disclaimer: Parts of this article may be copied verbatim or almost verbatim from the referenced research paper.
References
- Iván Rivilla, Borja Aparicio, Juan M. Bueno, David Casanova, Claire Tonnelé, Zoraida Freixa, Pablo Herrero, Celia Rogero, José I. Miranda, Rosa M. Martínez-Ojeda, Francesc Monrabal, Beñat Olave, Thomas Schäfer, Pablo Artal, David Nygren, Fernando P. Cossío & Juan J. Gómez-Cadenas (2020) Fluorescent bicolour sensor for low-background neutrinoless double β decay experiments Nature doi: 10.1038/s41586-020-2431-5 ↩
1 comment
[…] konplexuagoa da, eta, esaterako, argibide teknikoagoak nahi dituenak, Cesar Tome Lopezek idatzitako azalpen honetan aurki […]