How does a fly smell? Asymmetrically!

Smell has often been the neglected sense, despite—or, hopefully, until—the increasing number of interesting discoveries being made about and around it. Trivially, smells are interpreted as a series of neurochemical reactions mediated by receptors; this is no novelty, and at the single-molecule and single-neuron level the mechanism (how a molecule triggers a specific receptor which in turn fires a set of neurons, provoking a physiological response) is fairly understood. But, as usual when neurons are involved, the complexity of neural arrays makes understanding of the process at the organ or even organism level a daunting one.
So, what do you do when you can see what happens inside a neuron but going all the way to what happens in the brain proves a bit too complicated?
You use animal models, of course. You work the process out in a relatively simpler organism, like a leech or a fly, and wonder about the questions that could affect us. How does smell, for example, affect behaviour?
The work of Quentin Gaudry, Elizabeth J. Hong, Jamey Kain, Benjamin L. De Bivort and Rachel I. Wilson, published in Nature last January 17 1, addresses a deceptively-silly-sounding question: when a fruit fly detects a desirable smell, what does it take for it to change direction towards it?
Apparently, not a lot.
In many insects, odour receptors are unilateral: that is, a signal coming from the right side is detected more strongly by the receptors that are present—and connect—just to that side.
Drosophila melanogaster, the unsung hero of genetics, is not like that. Most of its olfactory receptor neurons (ORNs, for short) project to both sides of the brain. So, how does the fly know where the smell comes from in this case?
To find out, the authors glued a fly’s neck to its body to stop it from turning its head (isn’t science pretty?) and put it on a spherical treadmill with two little tubes sending delectable smells—to flies, at least—to each antenna. They chose a strain of wild-type Drosophila melanogaster that showed a robust running behaviour, and let it choose its path. As expected, when nice, ripe-fruit smells came from the left, the fly turned left.
What happened inside the fly to make it turn left?
The authors made a variety of tests to measure exactly, at the single-cell level and (this is the cool bit) in vivo, the way the ORNs react to a stimulus.
What they fond out is that, first, the fly turns towards the stimulus mid-stride. Literally: it took less time for the fly to turn (70 milliseconds) than to complete a stride’s period (100 milliseconds). So whatever causes the lateralization is quite fast and efficient.
And the other thing they found was that the ipsilateral neurons of the bilateral pair, that is, the neurons that were on the same side of the body that the stimulus was coming from, reacted faster and more frequently than the contralateral ones (the ones on the opposite side), thus allowing the lateralizationbehaviour.

Figure 1a shows the bilateral projection of an ORN. The circle marks the synapsis to the sister projection neurons (PNs), which serve as a kind of “extension cords” that convey the signal to the rest of the fly. Since it’s symmetrical, and since both target cells are morphologically and functionally identical—sharing even cellular lineage and birth dates—, discrimination has to happen by other means.
In Figure 1b you can see what you get when you measure the spikes from the projection neurons (post-synaptic). When the signal (odour) starts, the ipsilateral neuron (green) reacts earlier and more frequently (with more spikes, see the enlarged segment) than the contralateral neuron (in black).
Let’s see it another way, with all the data points.

DM6 and DM1 are two different neuronal glomeruli to which the ORNs connect (each “likes” a certain odour better), and what they measured was the rate at which the projection neurons (that came after the synapsis, remember) fired when stimulated. If both sides reacted the same way, you’d expect to see the data points fall more or less along the dashed line, at a 45 degree angle.
The blue dots, if you’re curious, mean spontaneous firing (in absence of stimuli).
What the data show instead is a marked bias, in both glomeruli, towards the ipsilateral (same side as the stimulus) neurons. This means that they fire up more frequently than the contralateral ones, and thus direct the fly to walk towards that side.
But where does that faster rate happen? Does it happen in the ORN, or after the synapsis, in the PNs? To find out, the authors expressed a genetically-encoded calcium indicator called GCaMP3.0 in the ORNs (pre-synaptic). They measured how much signal they got when made the fly “smell” some yummy pentanoic acid (which glomerulus DM6 “likes” especially) and found the same asymmetry in the side of the brain ipsilateral to the antenna that was receiving the stimulus.
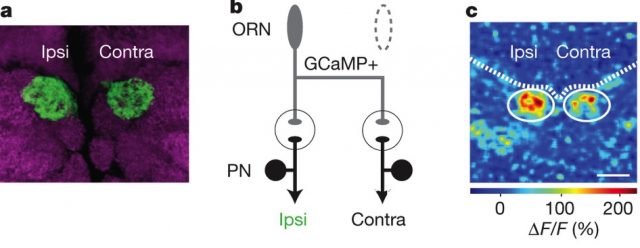
In both figure 3a and 3b, you can see how the ipsilateral side shows a more intense signal than the contralateral one. The signal can only be seen at the ORNs (because that’s where the marker is), before the synapsis, so it means the asymmetry happens there, and not after the synaptic gap.
The article proves that an asymmetry exists, but does not explain why, offering instead two possible explanations. One, that right and left sister neurons are not perfectly identical: they express different molecular tags, which could make them distinguishable. The other possible explanation has to do with the structure of an axon itself: it tends to have more release sites (for neurotransmitters) at proximal locations than at distal ones, and so it becomes a matter of distance: contralateral sites are more distal than ipsilateral ones.
The intriguing part of the work of Gaudry et al., however, is a question of degree: it’s not just that they proved the existence of an asymmetry; it’s that they quantified it and found that a mere 5% difference between ipsilateral and contralateral sides was enough to make a fly turn towards an odour. In their words: “[E]ven small signals in the Drosophila nervous system can be behaviourally relevant”. Thus, even though we still need to work out how to study increasingly complex neural arrays, relatively simple ones are giving us valuable clues that steer us along the causal chain that links pure biochemistry and the resulting behaviour in living, complex organisms.
References
- Quentin Gaudry; Elizabeth J. Hong; Jamey Kain; Benjamin L. de Bivort; Rachel I. Wilson. Nature 493, 424–428 ↩