Can you detect atom-size motion?
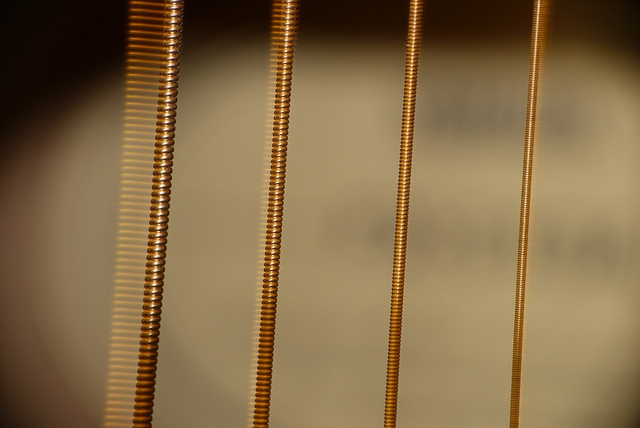
As you listen to music played in a guitar, you are getting much more than just plain sound. In fact, when the motion of the pulled strings translates into air waves, a big deal of information is carried along with the notes. You could say that mechanical properties of the musical instrument such as mass, displacement or forces are coded in the frequency of the sound wave. Unravel this information and you are tracing back properties of the guitar itself.
This concept is the essence of a technique to measure mechanical attributes of objects for which its small size challenges other conventional methods of measurement. To gain information, a mechanical mode of the object itself is transduced into another type of signal, such as light, which brings along properties describing the nature of the object. For that, light needs to be coupled to the resonant mechanical mode.
The use of light to decode a resonant frequency has, however, the disadvantage that the confinement of light is bottomed by the diffraction limit. That is to say that for objects in the diffraction-limit size and smaller, there is no place for light coupling, and thus no information can be conveyed out. For this reason, the technique is not applicable anymore for resonating cavities of approximately 200 nm and down, which nowadays is a huge size given the current technology development towards smaller sizes.
To overcome this limitation, researchers at FOM Institute AMOLF and Ecole Polytechnique Fédérale de Lausanne (EPFL) have used the strong confinement of the optical near fields in surface plasmons systems 1. In particular, they created a nanoscale cavity where, with the assistance of surface plasmons, the thermal motion was coupled to an optical far field.
Surface plasmons are oscillations that can exist at the interface between two materials. For the surface plasmon to exist, the real part of the dielectric function should change sign across the interface of the materials, such as occurring, for example, in a metal-air combination. The great attractive of surface plasmons for the technique described here is that they are bounded to the surface of the metal and as such, they are not diffraction-limited.
The researchers succeeded in coupling a mechanical vibrational mode of a cavity into surface plasmons. For their experiment, they fabricated a cavity consisting on two parallel bars of silicon nitride clamped at the edges to a silicon nitride membrane. In practice, due to mechanical tension in the membrane, the bars were suspended as if two long bridges next to each other on top of the membrane. These “bridges” had a length of 20 micrometers and a thickness of 50 nanometers each, and were separated by a small gap. To allow for several vibrational modes, the researchers fabricated several structures each with different widths of the gap between the two bars. The central gap between the two bars varied between 15 and 120 nm for the different structures.
Each of this tiny “bridges” could accommodate both out-of-plane and in-plane vibration modes of the bars, as illustrated in Figure 1. Thanks to the different gaps between the bars, the experimental setup contains a large range of vibrating bars, resembling miniature vowel cords vibrating at different frequencies.
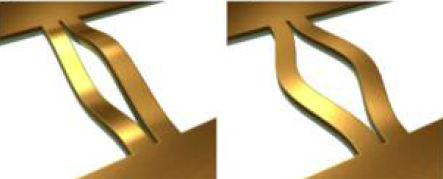
The silicon nitride bars are gold-coated on the side where they are facing each other. This gold coating plays a crucial role for the purpose of the experiment for it serves as means to couple light to the structure and sustain in this way the surface plasmon.
To excite the surface plasmons, a laser normal to the sample is focused on the slit between the two bars. The surface plasmons generated are confined in the gap between the golden coatings. These are called metal-insulator-metal (MIM) plasmons. The transmitted light is collected with a photodiode and converted to the frequency domain with a spectrum analyzer. To minimize the influence of the surrounding air on the thermal behavior of the sample, the experimental setup is located in a vacuum chamber pumped at 10-3 mbar.
The frequency measurements of the whole set of nanostructures showed the expected behavior of an increasing resonant wavelength for smaller widths of the insulator gap region. To assess that the signal is indeed mechanical in origin, the gas pressure in the chamber was systematically varied, resulting in a vanishing resonant signal for pressures above approximately 1 mbar.
The black curve in Figure 2 shows the signal measured for one of the cavities for a spectrum of frequencies. The peaks in the graph show four clear mechanical resonances. Using finite-element-method simulation, the team obtained the expected eigenfrequencies, which matched to the experimental findings. This allowed them to identify the corresponding modes of this double-bridge structure, which are both out-of-plane and in-plane oscillations (see the insets of Figure 2). Also in Figure 2, the grey curve shows the results for a nanostructure without gold coating between the bars. In this case, the absence of the metal prevents the surface plasmon excitation, and results in a flat spectrum.
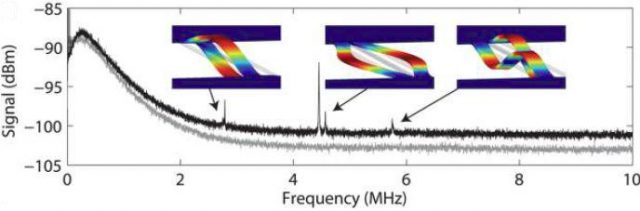
Once these experimental results testified of the existence of vibrational modes happening in the nanostructures, the researchers were ready to gain the desired mechanical information embedded in these signals. For that, the resonances curves are fitted to Lorentzian line shapes. From the fitting, one can determine properties such as the displacement of the bars during the vibration and the effective mass. The experimenters estimated that the little bridges were oscillating with displacement as small as 10.4 pm. In other words, the team of AMOLF and EPFL was able to build up an experimental setup that allowed them to detect movements in a size scale smaller than an atom.
This experiment opens the door into a type of sensors capable to probe atom-size objects. Not only new technologies using nanomechanical systems can benefit from this fast readout method of nanoscale mechanical motion, but it can also apply to a better understanding of biological systems, with applications for example for measuring mass of molecules.
References
- Thijssen R., Verhagen E., Kippenberg T.J. & Polman A. (2013). Plasmon Nanomechanical Coupling for Nanoscale Transduction, Nano Letters, 13 (7) 3293-3297. DOI: 10.1021/nl4015028 ↩