Polynuclear photocatalysts, a new generation?
Author: Daniel González-Muñoz is a predoctoral researcher in photocatalytic processes at Universidad Autónoma de Madrid.
One of the most important scientific objectives in recent years is to find solutions to the problem of climate change and environmental pollution. Unfortunately, this issue is not only related to the amount of CO2 that is released into the atmosphere from fossil fuels. The chemical industry, in the complex processes it carries out to synthesize products, generates a large amount of waste that is dumped into the environment. Imagine the process of synthesizing a drug to treat HIV, some type of cancer or a simple flu. The preparation of these drugs can require an enormous number of synthetic steps – 10, 20, 30 or even more – and each of these steps involves a multitude of reagents that make the process more expensive, while becoming potential pollutants released into the environment. That is why it is very important to develop chemical processes that require fewer steps and that the reagents used are as eco-friendly as possible. This way, we can protect the environment and chemical processes will become increasingly cheaper and profitable. At this point, the field of photocatalysis has gained enormous importance by using molecules (photocatalysts) capable of absorbing solar energy and transforming it into chemical energy. In this regard, the sun is the most abundant and accessible energy source on our planet and its application in the chemical industry will be a true disruption.
Although it appears to be a very original idea, applying it is not as easy as it seems. The solar spectrum covers the near ultraviolet (UV), visible region and infrared spectrum; specifically, 5% is UV radiation, 43% visible radiation and 52% infrared radiation (Figure 1) 1. The problem is that photocatalysts normally do not absorb in the entire solar spectrum, limiting themselves to UV radiation and part of the visible radiation, and this restricts their application with sunlight since only a very small part of the solar spectrum can be used. Therefore, it is vitally important to develop photocatalysts that absorb as much radiation as possible and absorb at wavelengths in the visible and infrared region, which is the most abundant.
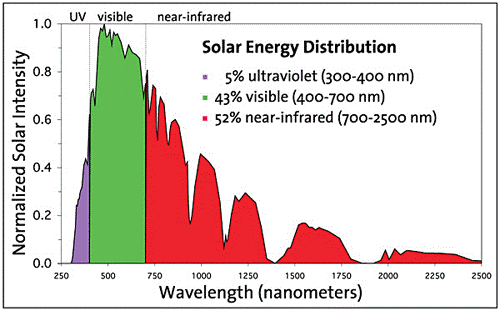
These characteristics can be covered by polynuclear photocatalysts 2 . As you can see in Figure 2a, a polynuclear photocatalyst can be obtained from a typical photocatalyst, [Ru(bpy)3]2+ by repeating its structure several times and obtaining molecules with 2 or more metallic centers. The absorption of the photocatalysts red-shifts towards longer wavelengths as the number of centers increases (Figure 2b). Given these changes in absorption wavelengths, the use of different light sources (white, blue, green or orange LEDs) can help evaluate the applicability and reactivity of each polynuclear photocatalyst. The emissions from the different light sources are also represented in figure 2b.
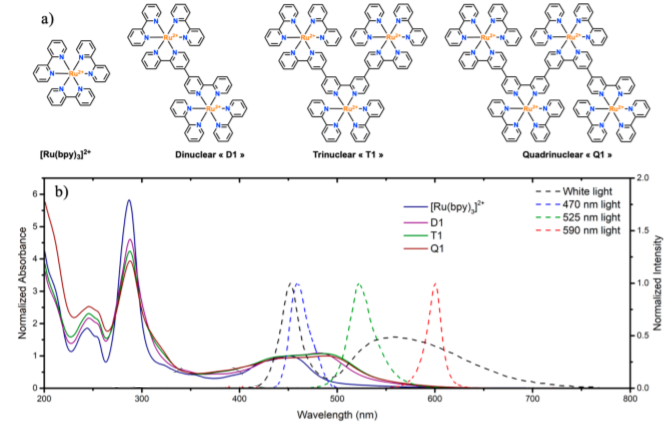
In addition to absorption spectra, other important properties can be studied in order to characterize a photocatalyst. The molar absorption coefficient (ε), which is the amount of light absorbed by the photocatalyst at a given concentration, can provide valuable information (Table 1). Mononuclear photocatalyst, [Ru(bpy)3]2+, has a high value, but as the number of centers increases, this value is even higher, reaching up to 54900 M-1 cm-1. In addition, when the light is absorbed by a photocatalyst, it goes into an excited state, and usually has a very short lifetime, in the order of nanoseconds. This property is measured with the excited-state lifetime (τ). These values are also represented in Table 1 and it is observed that the polynuclear photocatalysts have higher lifetimes, which means that they remain in the excited state longer and therefore, can be more reactive. The final property of photocatalysts we should discuss is the reduction potential to move from the excited state (Ru2+*) to a reduced species (Ru+), that is the ability of the photocatalyst to pick up an electron from another species (Table 1, E1/2). These values increase as the number of centers increases. This means that polynuclear photocatalysts are more oxidizing compounds, and as we will explain later, can lead to enhanced activity.
Photocatalyst | ε (M-1 cm-1) | τ (ns) | E1/2 (Ru2+*/+) (V) |
[Ru(bpy)3]2+ | 11900 (450) | 1020 | 0.83 |
D1 | 21800 (480) | 1920 | 0.98 |
T1 | 40100 (485) | 1580 | 1.01 |
Q1 | 54900 (490) | 1470 | 1.10 |
Table 1. Molar absorption coefficients (values in parentheses indicate the corresponding wavelength in nm), excited-state lifetime and reduction potentials of the ruthenium photocatalysts.
The polynuclear photocatalysts were evaluated in the dehalogenation reaction represented in figure 3a where the chloride functionality present in 1 is reduced to form 2. The reaction under white light illumination (figure 2b, black dashed line) led to a 77% yield with [Ru(bpy)3]2+, whereas the use of polynuclear photocatalysts increased the reaction yields to >90%. Then, the reaction was studied with more specific wavelength light sources. With blue and green light (figure 2b, blue and green dashed lines respectively) the reaction yielded similar results to white light irradiation, which is not surprising considering the spectral overlap between the light emission profile and the absorption spectra of the photocatalysts. The most surprising results were obtained by using orange light (figure 2b, orange dashed line). [Ru(bpy)3]2+ photocatalyst led to a 31% yield of 2 while polynuclear photocatalysts reached >90% yield. To further demonstrate the potential of these photocatalysts, their catalytic amount in the reaction was reduced 25-fold. Using a white light, the reaction yield of 2 using [Ru(bpy)3]2+ was 66%, while polynuclear photocatalysts increased the reaction yield to around 90%.
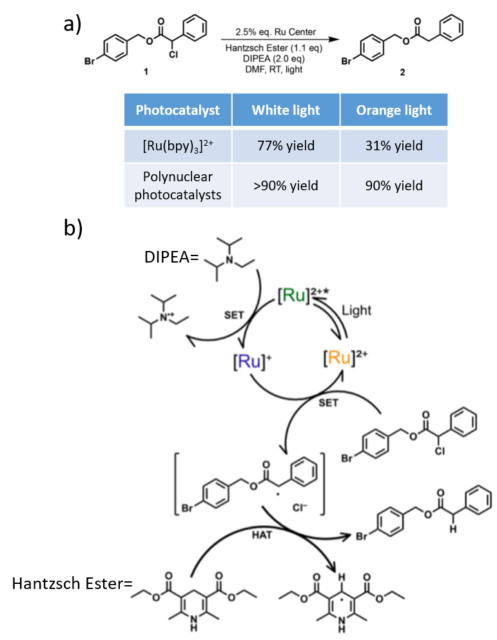
Additionally, a study was carried out in which it was verified that under irradiation of white light, ligand-loss photochemistry was observed for [Ru(bpy)3]2+ while polynuclear photocatalysts ligands remained stable.
The reaction mechanism is depicted in figure 3b. [Ru]2+ is excited with light to form [Ru]2+*, and this species takes an electron from DIPEA to form [Ru]+ and DIPEA·+ in a single-electron transfer (SET) event. At this stage, the value of the reduction potentials mentioned above is important. Polynuclear photocatalysts have higher values, which means that they will be able to pick up an electron from DIPEA more easily. Then [Ru]+ reduced the product 1 to form the radical intermediate and the recovered photocatalyst [Ru]2+. Finally, the Hantzsch Ester completes the hydrogen atom transfer (HAT) to the radical intermediate to form the final product 2.
In conclusion, polynuclear photocatalysts present a red-shift absorption, better molar absorption coefficient (ε) and longer excited-state lifetime (τ). They are better oxidants and present a better ligand stability in comparison with mononuclear photocatalyst [Ru(bpy)3]2+. All these properties translate into better photocatalytic activity in the dehalogenation reaction. These results are very promising since they can widen the possibilities to develop more industrially effective photocatalysts and that solar radiation can be used effectively for this type of process.
References
- Dario Cambié, Timothy Nöel (2018) Solar Photochemistry in Flow. Top. Curr. Chem., 376, 45-72. doi: 10.1007/s41061-018-0223-2. ↩
- Simon Cerfontaine, Sara A. M. Wehlin, Benjamin Elias, Ludovic Troian-Gautier (2020) Photostable Polynuclear Ruthenium(II) Photosensitizers Competent for Dehalogenation Photoredox Catalysis at 590nm. J. Am. Chem. Soc. 142, 5549-5555. doi: 10.1021/jacs.0c01503 ↩
2 comments
[…] interes ekonomiko eta ingurugiro interesa du. Hona fotokatalizatzaile polinuklearren garrantzia: Polynuclear photocatalysts, a new generation? Daniel González-Muñozen […]
[…] Latest Generation of Plynucle […]