Materials for caging the Sun on Earth
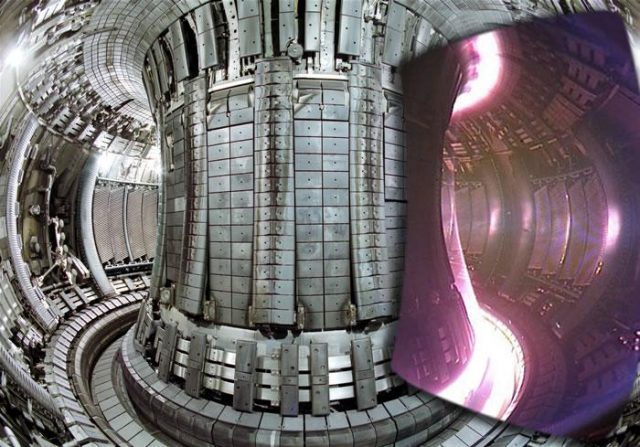
The most widely used metaphors for nuclear fusion reactors are the recreation of the Sun on Earth and the controlled explosion of a hydrogen bomb. In both cases, the first thing that comes to mind is the need for high-performance radiation-resistant materials to contain the fusion reaction. The selection and design of new materials that will withstand the extreme conditions of a fusion power plant requires significant scientific advances in our understanding of the process of radiation damage by high-energy neutrons.
One of the main sources of severe damage of metals and alloys is the embrittlement of helium atoms product of the transmutation of neutrons. Sergio L. Palacios (University of Oviedo, Spain) and colleagues have developed the very first ab initio simulation of helium clustering in copper by using a density functional theory simulator 1. Let me summarizes their results which can be incorporated into multiscale modelling codes for the design of new innovative materials based on copper alloys to be used in the future commercial fusion reactors.
The most promising technology for fusion reactors is the tokamak, a toroidal-shaped vessel confining a high-temperature ionized plasma by means of powerful toroidal and poloidal field magnets. The fusion reaction between deuterium and tritium (D-T) produces heat and energetic neutrons to be absorbed by the surrounding first wall and blanket structural materials. Current estimates of the steady-state heat fluxes for first-wall components will be in the range of 1 to 10 MW/m2, substantially higher than the highest heat flux for structural materials in fission reactors (about 1 MW/m2 for the fuel cladding) 2.
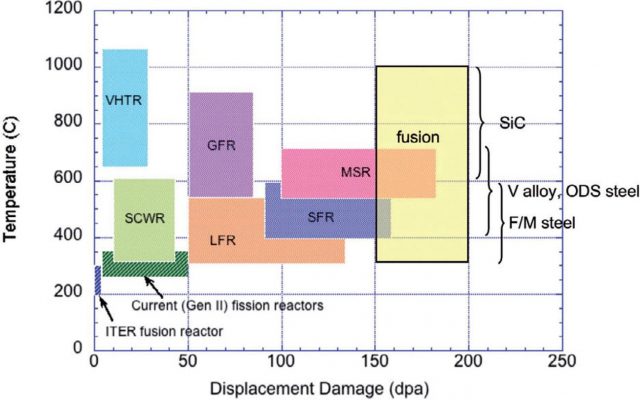
The energetic neutrons produced by the fusion reactions can dislodge a large amount of atoms from their lattice sites over the operating lifetime of the reactor. The most common defects in the bulk material can be classified as both vacancies, missing lattice atoms, and self-interstitial atoms (SIAs), atoms residing in the lattice interstices. The radiation damage is usually quantified in terms of displacements per atom (dpa): the number of displaced (temporarily or definitively) atoms per target atom in the solid, induced by bombardment with ionizing radiation.
At very high temperatures, larger than a half of the melting temperature of the material, and under applied mechanical stress, helium produced by neutron transmutation reactions in the structural material may migrate to grain boundaries and form cavities. The formation (nucleation) and growth of helium bubbles results in embrittlement and causes severe damage. The first-wall materials of the ITER fusion reactor have been designed for less than 5 dpa; however, for commercial fusion reactors the damage could be in the range of 10 to 200 dpa [2]. In fact, this is the major factor limiting the maximum allowable operating temperature in a fusion reactor.
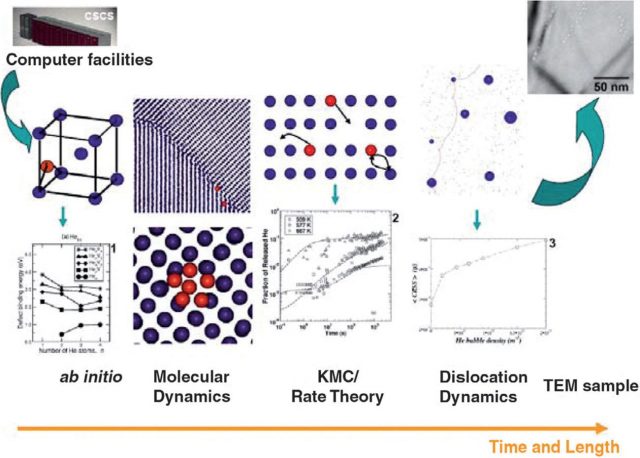
The understanding of the dynamical processes involved in helium bubble nucleation, growth and migration requires a multiscale computational approach coupled with validation experiments 3. At the lowest level, ab initio quantum simulations are implementedby means of the density functional theory (DFT). At the next levels, molecular dynamics (MD), kinetic Monte-Carlo (KMC), rate theory (RT), and dislocation dynamics (DD) techniques are used. For validation, the results must be compared with the measurements obtained from thermal He desorption spectrometry (THDS) and transmission electron microscopy (TEM).
Density functional theory is the most widely used atomic-scale model for nanoscale defects produced by irradiation and their short-range interactions 4. The defects are treated as quasi-particles characterized by their position, velocity, mass, and effective mobility. They can migrate, react, coalesce, and grow inside the atomic lattice of the material. Most DFT calculations were carried out by using well-documented DFT computer programs. González et al [1] used the commercial software VASP (The Vienna Ab initio Simulation Package), which computes an approximate solution to the many-body Schrödinger equation. Let me emphasize that there are other DFT codes using similar approaches like CASTEP, SIESTA, PLATO, PWSCF, and ABINIT 5.
Copper has been proposed as structural material for the first-wall in fusion reactors due to its relatively high melting temperature (1084 °C) and its immiscibility with most other elemental metals 6. Bulk copper has a face-centered cubic (fcc) unit cell. In the simulations of González et al. [1] the defects are studied in a 4×4×4 cubic supercell composed of 256 Cu atoms. Such a supercell allows up to four vacancies and the incorporation of up to four He atoms, both in the the pure structure and/or combined with the Cu vacancies.
To validate their approach, González et al. [1] first consider vacancy and SIA defects, comparing their results with others found in the literature. The most stable structures in the mono-, di-, tri-, and tetra-vacancy cases are discovered by minimization of the formation energy. After the validation of their methodology, they consider the He bubble formation inside the perfect bulk and the vacancy defects.
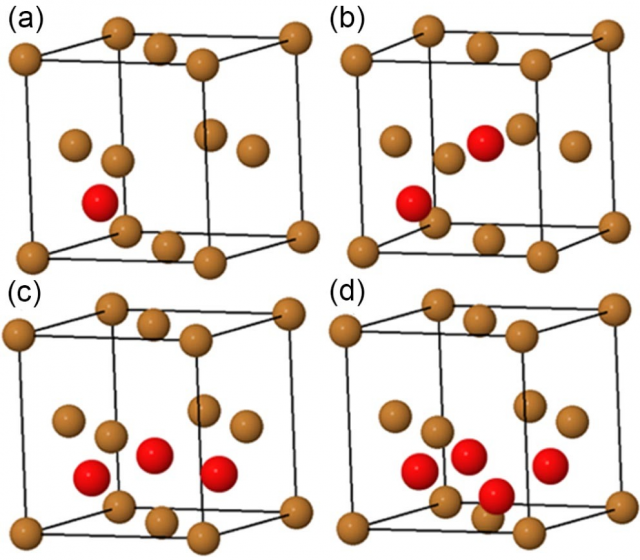
The most noticeable phenomenon observed in the simulations is the remarkable mobility of the helium atoms inside the vacancies. The first He atoms occupy the empty place in the mono-vacancy case, the so-called substitutional position, but the center of the configuration in the multi-vacancy cases. The addition of He atoms result in a reconfiguration of the geometrical structure of all the He atoms in the bubble. Hence, an increase of the formation energy (almost) linearly with the number of He atoms forming the bubble is observed. Furthermore, the most favourable point defects for He clusters to nucleate and grow are those with the largest number of vacancies.
Let me emphasize that the work of González et al. [1] has limitations to be solved by further studies. The most obvious one is the need for larger supercells capable to deal with larger He bubbles (but also requiring larger computational resources). Another one is the search for a possible saturation of the formation energy for large enough bubbles. This phenomenon, if it exists, will be very useful for the design of first-wall, plasma-facing, and diagnostic materials based on copper.
In summary, the binding and formation energies of He bubbles calculated by González et al. [1] are a useful input for other techniques undertaking a multiscale modelling of the migration, coalescence, and growing of He bubbles inside the atomic lattice of metallic copper. Let me finish with a remark of Duffy’s review [6]: “The challenges involved in designing materials for an economically viable fusion power plant are formidable, but none of the perceived obstacles should be insurmountable.”
References
- González C., Fernández-Pello D., Cerdeira M.A., Palacios S.L. & Iglesias R. (2014). Helium bubble clustering in copper from first principles, Modelling and Simulation in Materials Science and Engineering, 22 (3) 035019. DOI: 10.1088/0965-0393/22/3/035019 ↩
- Steven J. Zinkle and Jeremy T. Busby, “Structural materials for fission & fusion energy,” Mater. Today 12: 12-19, Nov. 2009. DOI: 10.1016/S1369-7021(09)70294-9 ↩
- Maria Samaras, “Multiscale Modelling: the role of helium in iron,” Mater. Today 12:46-53, Nov. 2009. DOI: 10.1016/S1369-7021(09)70298-6 ↩
- S. L. Dudarev, “Density functional theory models for radiation damage,” Rev. Mater. Res. 43: 35-61, 2013. ↩
- Available Electronic Structure Codes ↩
- D. M. Duffy, “Fusion power: a challenge for materials science,” Phil. Trans. R. Soc. A 368: 3315-3328, June 2010. DOI: 10.1098/rsta.2010.0060 ↩
1 comment
[…] sortu ditzaketen kalteen ulermena eskatzen du lehenik. Horretaz dihardu Francisco R. Villatorok Materials for caging the Sun on Earth […]