Quantum Physics + Genetic Engineering = Enhanced Energy Transport
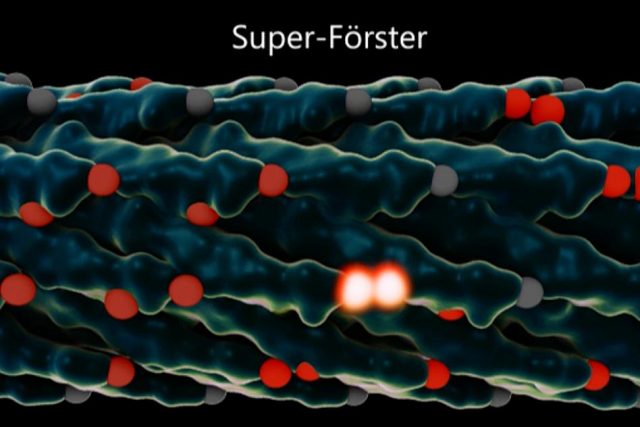
A hot topic of research nowadays is the energy transfer in quantum systems. One important reason for this interest is the famous 2007 experiment that suggests quantum effects in the dynamics of the photosynthetic complex FMO, from the green sulfur bacteria 1. This complex plays a crucial role in natural photosynthesis transferring an excitation from the antenna, where the sunlight is collected, to the reaction center, where the photosynthetic process takes place. It is well known that the efficiency of this process is considerable high, almost 100%, and it is very complicated to achieve such efficiency in an artificial light-harvesting system. Furthermore, the quantum nature of the process is also counterintuitive. In real conditions, these complexes are not isolated from the environment, and they work at room temperature 2. This implies that quantum effects should be cancelled by the environment in a very short timescale, but the experiment showed that they survived long enough to play a role in the energy transfer. Hence, we have an anomalous high efficiency and an anomalous quantum behavior. The combination of these two unexpected phenomena gives rise to a question: Is this process efficient because it is quantum?
It is difficult to exaggerate the importance of understanding this process. The efficiency of many physical systems, from computers to solar cells, can improve dramatically if we learn how to harness their quantum properties. Very recently, a multidisciplinary team has achieved one milestone in this direction. This group performed an experiment combining genetic engineering with quantum transport. In this work, recently published in Nature Materials 3, the researchers used a genetically modifiable virus template to create a tunable light harvesting material. They created two types of viruses, M13CF with a uniform distribution of weakly coupled chromophores and M13SF with clusters of strongly coupled chromophores (See figure 2).
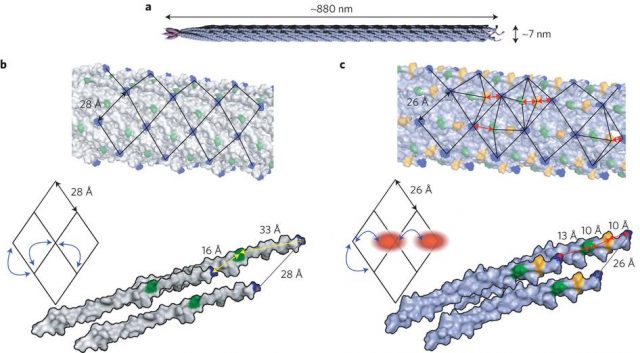
Theoretical calculations predict that the weakly-coupled network exhibits a semi-classical (Förster) dynamics and the strongly-coupled presents a combination of both classical and quantum dynamics (super-Förster). Unfortunately, performing direct measures of the energy transfer in this kind of system is beyond nowadays technologies. Hence, instead of a direct measure the researchers have to rely on indirect spectroscopy analyses. The researchers performed this analysis by measuring the fluorescence of the system under illumination. The viruses contain acceptor chromophores that emit energy if they receive an excitation. They also include donor chromophores, which can absorb light from a definite wavelength. When the system is under illumination the following process takes place: Donors absorb photons from the radiation and this absorption creates excitations in the system. These excitations can travel around by interacting with other chromophores. Finally, if an excitation arrives at an acceptor some light is emitted. Both donors and acceptors are distributed around the virus and by measuring the intensity of the fluorescence we can measure the number of excitations that arrive at an acceptor. The fluorescence constitutes a measure of the diffusion length of the excitation, as an excitation that propagates through a higher distance has a higher probability of finding an acceptor. In figure 3 a scheme of the process, together with the fluorescence results for both viruses with different values of the number of donors and acceptors.
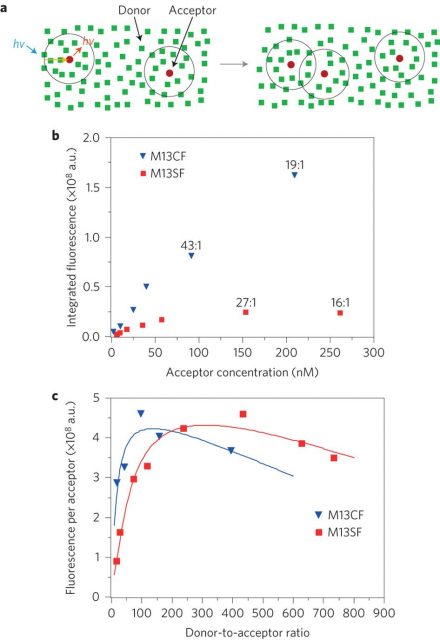
Numerical calculations based on the fluorescence results yield the conclusion that the excitonic diffusion length of the strongly coupled virus is a 68% longer than the weakly coupled one. A different question is if the transport is really quantum in the high efficient case or it is not. Again, a direct measure is very difficult to perform but a numerical comparison is possible. The researchers used different models of transport and applied them to the virus topology, in order to theoretically explain the empirical data. Due to the lack of full knowledge about the system variables it is very difficult to really fit the experimental data with theoretical models, but as it is shown in Figure 4 the Super-Förster theory is the only one that can predict the qualitative behaviour of the system.
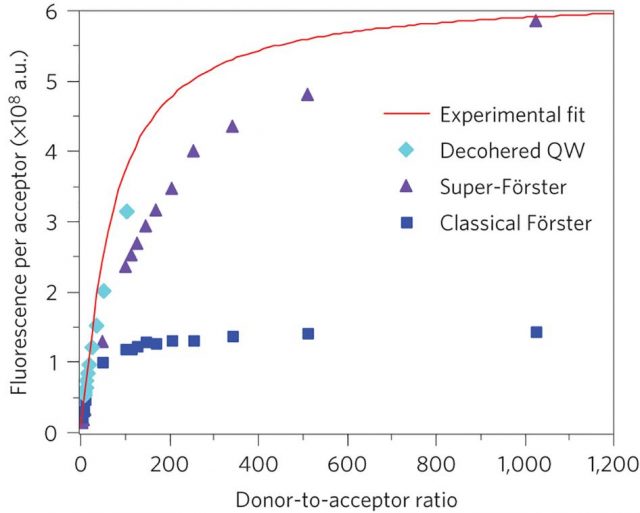
This research is very important as it combines very different techniques to handle an interesting and timing problem. It is also interesting from both theoretical and applied point of views. The question of the efficiency enhancement due to quantum effects is on the table. These results do not prove this hypothesis, but they add more evidence in this direction. From the applied perspective, this experiment opens so many possibilities that it is impossible to discuss them all.
References
- G.S. Engel et al. Evidence for wavelike energy transfer through quantum coherence in photosynthetic systems. Nature 446, 782 (2007). ↩
- The first experiment was performed at ultralow temperatures, but the same effect was found at room temperature, see E. Collini et al. Coherently wired light-harvesting in photosynthetic marine algae at ambient temperature. Nature 463, 644 (2010). ↩
- Heechul Park et al. Enhanced energy transport in genetically engineered excitonic networks. Nature Materials. DOI:10.1038/NMAT4448. (2015). ↩