Pulsed radiotherapy
Author: Álvaro Peralta Conde is a senior researcher at CLPU (Pulsed Lasers Centre)
Can radiotherapy with laser-produced ionizing radiation be an alternative to conventional radiotherapy? Can we deep our understanding of the basic mechanisms of radiation damage? Can we study the very early biological response of the living matter and use this knowledge to design more efficient radiotherapy treatments?
Contrary to popular belief, when tumoral tissue is irradiated the DNA damage that may lead to cell death is mostly produce – especially for low LET radiation1 – by the energy deposition pattern of the radiation through the cell, rather than by a direct interaction with the DNA. It is true that ionizing radiation can eventually produce directly single and double strand breaks (SSB/DSB) on the DNA –direct damage-, but these lesions are very localized and the repair cellular mechanisms rejoin them efficiently, increasing the survival rate of the cells 123456. However, the interaction of ionizing radiation with the cellular medium induces different phenomena in the molecular constituents, being the most relevant the creation of free radicals via water radiolysis. Free radicals are highly unstable molecules capable to transfer chemical damage to the cell constituents including the DNA –indirect damage-. In contrast to direct damage, for indirect damage the initial ionizing track through the cellular medium defines the spatial distribution of defects in the DNA. That means, a cluster of SSB and DDB lesions is produced 78910. For such DNA clustered damage the repair rate is low, defining hence the fate of the tumor cells (see Fig. 1).
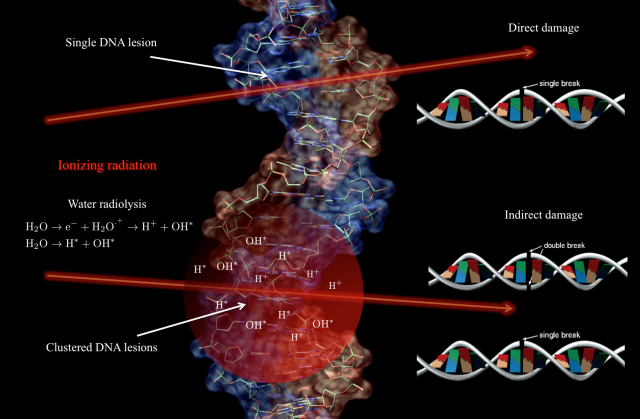
In this context, and aiming to design new and more efficient radiotherapy treatments and protocols, it is an important step forward to understand two main closely related issues: on one hand, the radiobiology at dose-rates higher than the currently used typically of 10 Gy/s 1112 2, and on the other hand, we must expand our limited knowledge of the molecular dynamics of the irradiated cells and obtain a clear temporal picture of the cellular processes triggered by the radiation. So far it is not known if delivering the same dose but with an ultrahigh instantaneous dose-rate can trigger different reactions in the tumoral cell with therapeutic consequences. It is plausible to think that high fluxes can induce nonlinear or collective effects that cannot be described by the sum of the effect of independent particles. Moreover with the current treatments the energy deposition overlaps the primary creation of radicals, the biomolecular damage (membranes and DNA lesions), and the onset of cell repair mechanisms (see Fig. 2). Although this temporal overlapping does not affect in principle the efficiency of tumor regression, it may complicate the biological processes involved in DNA repair on for example the surrounding healthy tissue 131415.
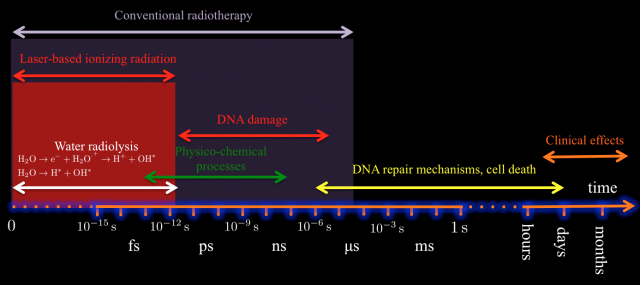
Luckily, we have already developed all the tools required for answering such questions. In fact, picosecond/femtosecond particle bunches with dose rates exceeding 109 Gy/s are routinely generated in laser laboratories, expecting to increase by orders of magnitude this limit in a near future 161718192021 (see Fig.3 for one of the possible setups of these sources). These values contrast with those provided by conventional radiotherapy treatments with dose rates in the order of 10 Gy/s and bunches of microseconds time duration. However, so far in literature one can find only few preliminary studies with different cellular lines and mostly in vitro, showing inconclusive results (see for example 2223242526). This is odd given the increasing interest of a broad community -nuclear physicists, biologists, radiologist, medical doctors…- for the potential impact of this technology.
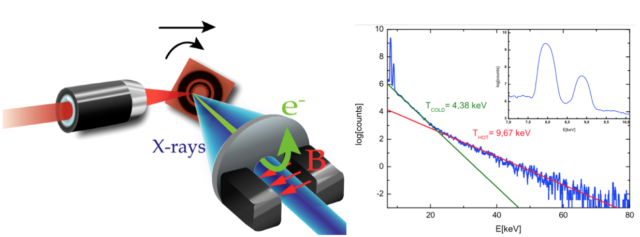
Last but not least, it is important to mention the economical aspects of these studies. In 2012 the global market for radiation therapy devices was about 4000 millions in 2017 growing at an annual rate of 5.4% from 2013 to 2017. It is a continuously growing market feed by novel techniques and patient treatment protocols. Thus, there is a clear industrial interest in the development and commercialization of new techniques to become more competitive and profitable. Also, the hypothetical appearance of laser-based radiotherapy will reduce the size and complexity of the required equipment with a reduction in the economical cost of the treatments and obvious benefits for institutions and patients.
At this point and bearing in mind the profound implications of this research, it is important to be absolutely honest. These ideas constitute an ambitious attempt to establishing a new framework at the frontier of biology, physics, chemistry, and medicine requiring of multidisciplinary teams and unique approaches. But there is a certain possibility that such laser-based ionizing radiation offer no benefit when compared with the current radiotherapy methods. It is a high risk-high reward research. Do we believe -as a society- that it is worth to invest resources and time in exploring these ideas? This is the first question we need to answer.
Notes:
1 LET is the acronym for Linear Energy Transfer and can be understood as the amount of energy deposited by radiation per unit length of travel. Protons and α- particles are high LET radiation while electrons and X-rays are low LET.
2 The gray (symbol Gy) is defined as the absorption of one joule of radiation energy per kilogram of matter.
References
- T. E. Schmid, G. Dollinger, W. Beisker, V. Hable, C. Greubel, S. Auer, A. Mittag, A. Tarnok, A. A. Friedl, M. Molls, and B. Röper, Int. J. Radiat. Biol. 86, 682 (2010). ↩
- A. Asaithamby, N. Uematsu, A. Chatterjee, M. D. Story, S. Burma, and D. J. Chen, Radiat. Res. 169, 437 (2008). ↩
- P. A. Jeggo and M. Lobrich, Cell Cycle 4, 359 (2005). ↩
- B. Jakob, J. Splinter, S. Conrad, K. O. Voss, D. Zink, M. Durante, M. Löbrich, and G. Taucher-Scholz, Nucleic Acids Res. 39, 6489 (2011). ↩
- P. Reynolds, J. A. Anderson, J. V. Harper, M. A. Hill, S. W. Botchway, A. W. Parker, and P. O’Neill, Nucleic Acids Res. 40, 10821 (2012). ↩
- T. Helleday, E. Petermann, C. Lundin, B. Hodgson, and R. A. Sharma, Nat. Rev. Cancer 8, 193 (2008). ↩
- M. E. Lomax, L. K. Folkes, and P. O’Neill, Clin. Oncol. 25, 578 (2013). ↩
- J. Nguyen, Y. Ma, T. Luo, R. G. Bristow, D. A. Jaffray, and Q.-B. Lu, Proc. Natl. Acad. Sci. U. S. A. 108, 11778 (2011). ↩
- M. D. Coltrera, The Basic Science of Oncology (MacGraw Hill, 1992). ↩
- P. Mayles, A. Nahum, J. C. Rosenwald, and N. Papanikolaou, Handbook of Radiotherapy Physics: Theory and Practice (Taylor & Francis, 2008). ↩
- M. Durante and J. S. Loeffler, Nat Rev Clin Oncol 7, 37 (2010). ↩
- A. Giulietti, Laser-Driven Particle Acceleration Towards Radiobiology and Medicine (Springer, 2016). ↩
- D. Caldwell, Int. J. Quantum Chem. 9, 755 (1975). ↩
- R. D. Cooper and R. W. Wood, Physical Mechanisms in Radiation Biology: Proceedings of a Conference Held at Airlie, Va., Oct. 11-14, 1972, Sponsored by the U.S. Atomic Energy Commission, Division of Biomedical and Environmental Research (Technical Information Center, Office of Information Services, United States Atomic Energy Commission, 1974). ↩
- F. M. Khan, Physics of Radiation Therapy Third Edition (Lippincott Williams & Wilkins, 2003). ↩
- H. Daido, M. Nishiuchi, and A. S. Pirozhkov, Rep. Prog. Phys. 75, 56401 (2012). ↩
- S. Corde, K. Ta Phuoc, G. Lambert, R. Fitour, V. Malka, A. Rousse, A. Beck, and E. Lefebvre, Rev. Mod. Phys. 85, (2013). ↩
- A. Macchi, M. Borghesi, and M. Passoni, Rev. Mod. Phys. 85, 751 (2013). ↩
- F. V. Brozas, A. V Carpentier, C. Salgado, J. I. Apiñaniz, M. Rico, M. S. Albaneda, J. M. Álvarez, A. P. Conde, and L. Roso, Rev. Española Física 29, 3 (2015). ↩
- F. Valle Brozas, A. Crego, L. Roso, and A. Peralta Conde, Appl. Phys. B 122, 220 (2016). ↩
- F. V. Brozas, D. Papp, L. M. Escudero, L. Roso, and A. Peralta Conde, Sumitted to Appl. Phys. B; https//arxiv.org/abs/1702.01022 (2017). ↩
- R. Meesat, H. Belmouaddine, J.-F. Allard, C. Tanguay-Renaud, R. Lemay, T. Brastaviceanu, L. Tremblay, B. Paquette, J. R. Wagner, J.-P. Jay-Gerin, M. Lepage, M. A. Huels, and D. Houde, Proc. Natl. Acad. Sci. 109, E2508 (2012). ↩
- X. Kong, S. K. Mohanty, J. Stephens, J. T. Heale, V. Gomez-Godinez, L. Z. Shi, J. S. Kim, K. Yokomori, and M. W. Berns, Nucleic Acids Res. 37, (2009). ↩
- A. Yogo, T. Maeda, T. Hori, H. Sakaki, K. Ogura, M. Nishiuchi, A. Sagisaka, H. Kiriyama, H. Okada, S. Kanazawa, T. Shimomura, Y. Nakai, M. Tanoue, F. Sasao, P. R. Bolton, M. Murakami, T. Nomura, S. Kawanishi, and K. Kondo, Appl. Phys. Lett. 98, 53701 (2011). ↩
- O. Rigaud, N. O. Fortunel, P. Vaigot, E. Cadio, M. T. Martin, O. Lundh, J. Faure, C. Rechatin, V. Malka, and Y. A. Gauduel, Cell Death Dis. 1, e73 (2010). ↩
- C. Tillman, G. Grafström, a C. Jonsson, B. a Jönsson, I. Mercer, S. Mattsson, S. E. Strand, and S. Svanberg, Radiology 213, 860 (1999). ↩