Effects of defects and water on perovskite solar cells
The German mineralogist Gustav Rose (1798 – 1873) made important contributions in the fields of petrology and crystallography. He was the first to use a reflective goniometer in Germany and developed a particular interest in the relationship between the crystalline form and the physical properties of minerals. As a consequence, he contributed significantly to the creation of a mineral system that was a combination of chemistry, isomorphy, and morphology.
He identified many minerals new to science, including perovskite, that he found in the Ural mountains during an expedition throughout Imperial Russia. He named it in honor of Russian mineralogist Lev Aleksevich Perovski (1792–1856).
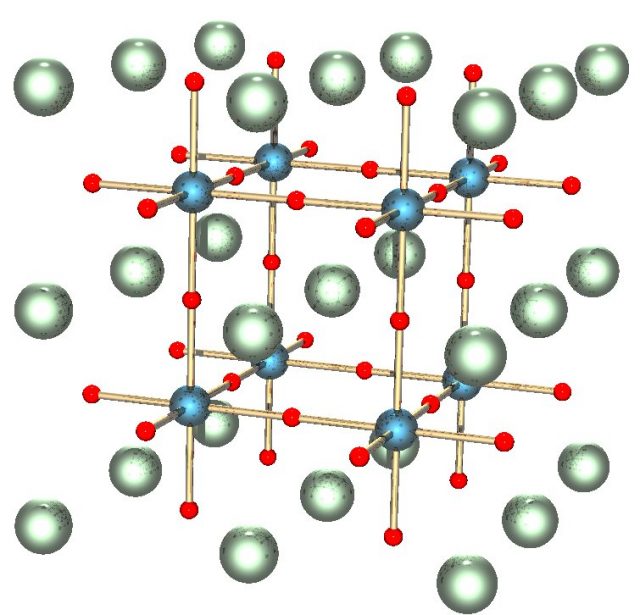
Perovskite lends its name to the class of compounds which have the same type of crystal structure as CaTiO3 (in general, ABX3, where A and B are cations) known as the perovskite structure. The perovskite crystal structure was first described byVictor Goldschmidt in 1926.The structure was later published in 1945 from X-ray diffraction data on barium titanate by Helen Dick Megaw.
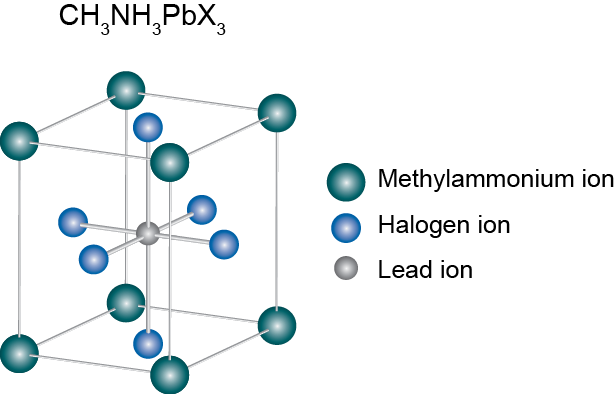
Perovskite structure remained only as a tool for inorganic chemists and crystallographers to help them understand compounds: just a name, so to say. But in 2009 Kajima et al published a paper that would change everything. They found that two organometallic halide perovskite nanocrystals, CH3NH3PbBr3 and CH3NH3PbI3, efficiently sensitize TiO2 for the visible-light conversion in photoelectrochemical cells. Since then, the name perovskite has been associated to a new revolution in solar cells.
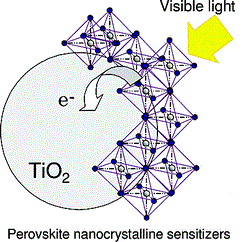
Perovskite materials such as the methylammonium (CH3NH3+ or MA for short) metallic halides are cheap to produce and simple to manufacture. Solar cell efficiencies of devices using these materials have increased from 3.8% in 2009 to a certified 20.1% in 2015, making this the fastest-advancing solar technology to date. According to a detailed balance analysis, the efficiency limit of perovskite solar cells is about 31%, which approaches the limit of gallium arsenide (33%). Their high efficiencies and low production costs make perovskite solar cells a commercially attractive option.
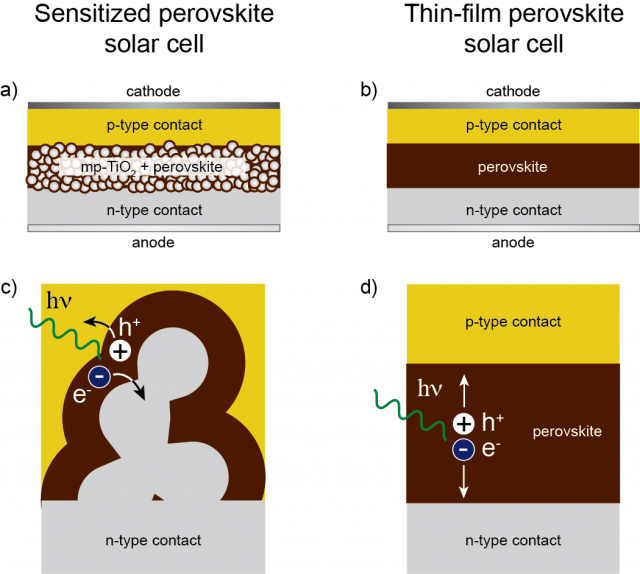
But, in order to increase the efficiency of perovskite solar cells some problems must be solved. For example, some general properties are still poorly understood and, as solar panels once installed are exposed to rain, it is necessary to know how water will interact with the perovskite and affect the efficiency. Jon Mikel Azpiroz, working at the Computational Laboratory for Hybrid/Organic Photovoltaics (CLHYO/ISTM-CNR) in Perugia (Italy) and DIPC, and his colleagues have significantly contributed to the theoretical understanding of perovskites behaviour in two recent papers.
Azpiroz et al 1 first tackle some unusual findings which have earned a lot of attention but lack a proper theoretical explanation. Namely (1) the slow electrical material response under light irradiation; (2) the anomalous hysteresis (the relationship between variables depends on whether they are increasing or decreasing) of the current–voltage (J–V) curves shown by various perovskite devices; and (3) a recently reported giant switchable photovoltaic effect.
On the one hand, a recent work has critically assessed the role of charge trapping and ferroelectricity as the source of the slow response and hysteresis. On the other, ion or defect migration could also explain the giant switchable photovoltaic effect as organohalide lead perovskites, with their propensity for defect formation, are potential candidates for ionic conductivity.
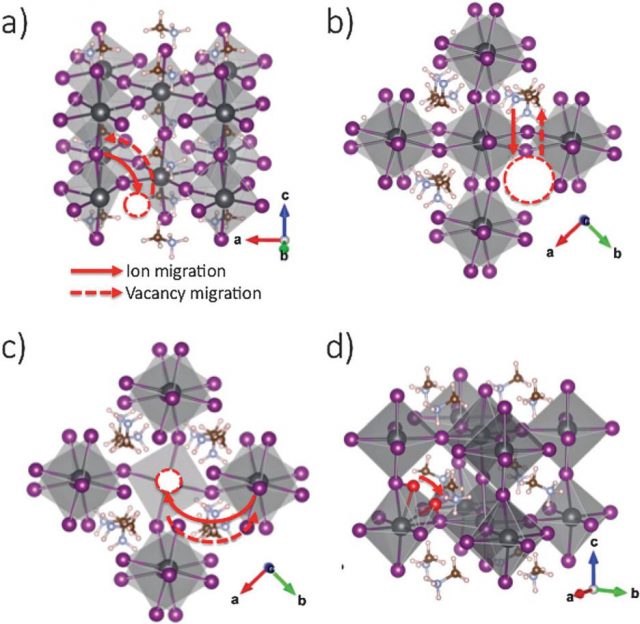
What Azpiroz et al do is a state-of-the-art first-principles computational analysis on realistic models of MAPbI3 and MAPbBr3 along with the crucial MAPbI3 interface with TiO2. They build on previous theoretical studies which identified the most probable defects occurring in MAPbI3 and evaluate their migration energetics in tetragonal supercells.
The researchers found that both iodine vacancies interstitials show a very low activation energy to migration, ~0.1 eV, followed in the order of increasing activation energy by migration of MA (~0.5 eV) and Pb (~0.8 eV) vacancies. According to their calculations, the slow response typical in MAPbI3 materials could be explained by the diffusion of MA or Pb vacancies.
The models are sufficiently accurate to disclose the preferential location of defects close to the perovskite/TiO2 interface and allow to set up a quantitative model explaining the effect of migrating defects in operative solar cell architectures: slow photoconductivity, hysteresis, and switchable photocurrent. The identification of the mobile defects in perovskite solar cells, their migration across the perovskite material, and their effect on the operational mechanism of the device, may pave the way for the development of new solar cells with improved efficiencies.
All is very well, but what happens to perovskites when the solar panels are wet because of moisture, dew or rain? This was the other problem that Azpiroz contributed to clarifying 2 recently.
Together with his colleagues, Azpiroz carried out ab initio molecular dynamics simulations to investigate the nature of the heterogeneous interface between water and the prototypical MAPbI3 perovskite and to analyze the degradation and solvation processes which the perovskite may undergo in the presence of liquid water, along with a characterization of the solvated species.
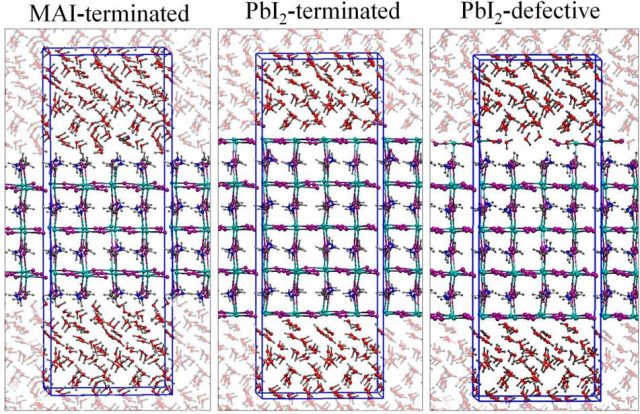
perovskite slabs. | Credit: Mosconi et al (2015)
The results show that the effect of water depends on the surfaces. Thus, MAI-terminated surfaces are easily prone to solvation, a process that is initiated by the nucleophilic substitution of dangling I ions on the surface by water molecules. Such a process is assisted by the solvation of neighboring MA cations at the same time.
The PbI2– terminated surface, characterized by shorter Pb−I bonds compared to the bulk, is found to be less sensitive to the presence of interfacial water, suggesting that the PbI2– terminated surface could act as a protective layer. But, in the case of (PbI2)n “vacancy” defects, which easily form under PbI2– poor conditions, they could lead to a substantially weakened PbI2-terminated surface.
Finally the researchers analyze the impact of hydration on the perovskite electronic structure, finding a general band gap increase upon perovskite interaction with water with almost no changes in the tetragonal structure of the perovskite crystal.
This work, unraveling the atomistic details of the perovskite/water interface, may inspire new interfacial modifications and device architectures with increased stabilities, which could facilitate the widespread uptake of perovskite solar cells.
All in all, when new solar cells based on perovskites become commercially available, contributing to fight climate change and providing economical electricity to impoverished isolated areas of the world, Jon Mikel Azpiroz (1986 – 2015) would be, somehow, there.
Ion Mikel Azpirotz Apezetxea recently passed away. His colleagues and friends at the Theoretical Chemistry Group at UPV/EHU and DIPC want to dedicate these lines to his memory.
Ion Mikel, good friend, you will be in our hearts forever.
Author: César Tomé López is a science writer and the editor of Mapping Ignorance.
References
- Jon M. Azpiroz, Edoardo Mosconi, Juan Bisquert and Filippo De Angelis (2015) Defect migration in methylammonium lead iodide and its role in perovskite solar cell operation Energy Environ. Sci. DOI: 10.1039/c5ee01265a ↩
- Edoardo Mosconi, Jon M. Azpiroz, and Filippo De Angelis (2015) Ab Initio Molecular Dynamics Simulations of Methylammonium Lead Iodide Perovskite Degradation by Water Chem. Mater. DOI: 10.1021/acs.chemmater.5b01991 ↩