Can 3D printing mark a turning point in tissue engineering?
Nowadays we are getting more and more accustomed to making use of new technologies shortly after they have been developed. We like the idea of immediacy and our wishes coincide well with the commercial interests of industry. Nevertheless, there is a field in which this immediacy is almost impossible: the medical sector. Unfortunately, there is still a long way to go from pure research to clinical application, a period which is called “from bench to bedside”.
As a result, we have to face up to the hard truth: technologies available at the moment could represent an absolute revolution in the field of biomedicine, but the crude reality is that they are not being given the necessary support, mainly due to both the limited commercial interest in long-term research investment and also to the complexity of the regulatory aspects in this field.
A perfect example of this situation was shown in a recently published review article, by Melchels et al. 1, in which they enlighten us about the amazing prospects that are about to appear in the field of tissue engineering thanks to the introduction of additive manufacturing, commonly known as 3D printing. This technology is likely to revolutionize the field of regenerative medicine in the next few years.
Additive manufacturing (AM) consists of creating a three-dimensional object layer by layer using computer-aided design (CAD) 2.This technology was first developed in the 80’s in order to facilitate and hasten the creation of prototypes in industry. Since then, several additive manufacturing techniques have been developed and improved, so that many different fields of product manufacturing have been able to benefit from these technologies (e. g., aerospace and automotive industry employs AM to create lighter structures). There are numerous advantages to be found in AM technologies compared with other kinds of manufacturing processes: they are fast, they allow the production of very precise geometries and they can be used with a wide range of materials. For instance, they can produce metal, polymeric or ceramic materials, either from powder, liquid or solid precursors.
In the field of regenerative medicine, the use of 3D printing could bring about a dramatic difference. The concept is simple: imagine that we could turn the digital image of any kind of implant, tissue or organ into a real body part, no matter what the complexity of its shape, the kind of materials it is made of or its function inside the body. Just a specific body part customized for an individual patient with all the necessary physicochemical, mechanical or degradation properties.
Of course, the materialization of this idea is not so simple. The aim of tissue engineering is to promote the repair or regeneration of damaged or diseased tissues (i.e., muscles, skin, bones, cartilage, blood vessels and so forth) by means of a combination of two components: a scaffold or matrix (acting as a support structure) and both living cells or biologically active molecules. The scaffold or matrix is supposed to allow the cells to migrate, growth or differentiate, as needed. Then, this support structure will remain in the body or will disappear by biodegradation.
Current technologies have managed to successfully obtain cell-free scaffolds showing considerable clinical success in repairing bones, cartilage or skin, to name a few. However, when cell-based therapies are needed, available technologies only allow manual production on a one-by-one basis. This production is highly time-consuming and economically and logistically unfeasible to achieve clinical application.
3D printing can change this picture by introducing the automation of the manufacturing process in cell-based therapies. This technology will allow the simultaneous deposition of cells and scaffolding materials in a computer-controlled production, thus allowing the creation of personalized implants. Consequently, 3D printing will put together two apparently contradictory concepts: automation of the manufacturing process and patient-specific treatments.
Moreover, although two-dimensional (2D) techniques have helped to achieve a significant body of knowledge on cell biology and biochemistry, there are several phenomena occurring in living cells that need a three-dimensional structure to occur. At the end of the day, working in 2D is a considerable simplification if we are trying to capture the essential processes of life. Vascularization, i.e., the creation of blood vessels and capillaries in living tissues, is a good example of a critical aspect in tissue engineering that must be solved in three dimensions.
Additive manufacturing starts by generating a computer model with the aid of 3D CAD software. At this stage we can take advantage of different medical imaging techniques, such as magnetic resonance imaging, X-ray computed tomography or bioluminiscence imaging. Then, this computer model is sliced into thin layers that will be reproduced into a physical 3D object by deposition of the appropriate biomaterials layer by layer. Different AM techniques have been used in the production of tissue engineering constructs, but undoubtedly, stereolithography (SL) is the most accurate and developed of all them. SL consists of the curing of a photosensitive polymer by means of irradiation with an ultraviolet (UV) laser. It can achieve accuracies similar to the size of a single cell, and it has been successfully employed to obtain tissue engineering constructs with encapsulated living-cells. However, its main drawback is the limitation in the materials that can be used, as it requires the use of photo-curable polymers.
Another promising AM technique is robotic dispensing of biomaterials, also called dispensing bioprinters. This technology is quite simple, it allows the deposition of highly viscous gels with encapsulated cells and they are versatile regarding the variety of materials that can be used, the dispensing mechanisms and the environmental conditions in which the manufacturing process takes place.
Together with the development of appropriate AM technologies for tissue engineering, another critical aspect waiting to be solved is the lack of adequate materials for the design and fabrication of scaffolds. Although many polymers and ceramics have been successfully processed by AM techniques, the required processing conditions (usually high pressure and temperature, use of solvents, etc) prevent from the use of living cells. Actually, most of these materials come from other areas of knowledge and they have not been developed for their application in the biomedicine field.
All in all, there are grounds for thinking that the application of additive manufacturing techniques in the field of tissue engineering, although still at an early stage, could mark a turning point in cell-based regenerative therapies. The automation of the fabrication process will allow high resolution and flexibility in design leading to versatile, reproducible and personalized implants. As is always the case, the scale-up of these technologies together with the development of new suitable biomaterials will be closely related to commercial interests, government funds and the progress made by regulatory agencies regarding cell-based therapies.
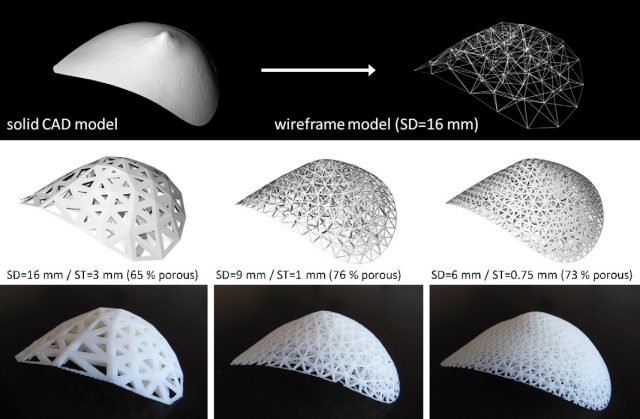
References
- Ferry P.W. Melchels, Marco A. N. Domingos, Travis J. Klein, Jos Malda, Paulo J. Bartolo, Dietmar W. Hutmacher, Additive manufacturing of tissues and organs, Progress in Polymer Science, Vol. 37, Issue 8 (2012) p. 1079. http://dx.doi.org/10.1016/j.progpolymsci.2011.11.007 ↩
- Kaufui V. Wong and Aldo Hernández, A review of additive manufacturing, ISRN Mechanical Engineering, Vol. 2012, Article ID 208760, 10 pages, 2012. doi: 10.5402/2012/208760. ↩
1 comment
Hi, I just stumbled upon this article. Thanks for spreading the word and making our work understandable to the general public. It may have looked like science fiction 11 years ago, but large strides have been made. The concept is being realised by https://www.bellaseno.com/; 36 patients have received 3D-printed breast scaffolds with success, with up to 2 years follow-up. They have started to treat other body areas as well. Kind regards, Ferry