The attosecond dynamics underlying the photoelectric effect
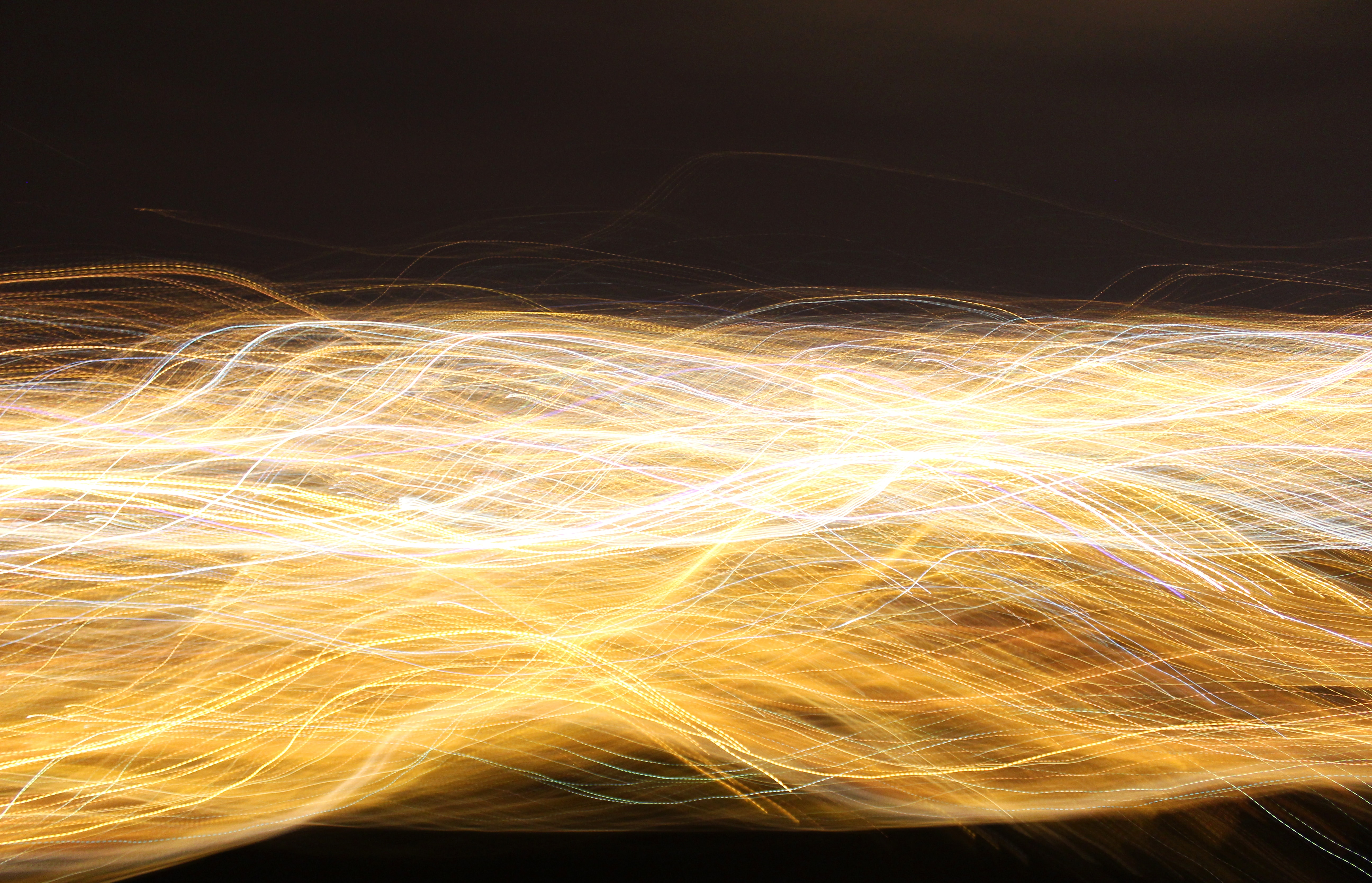
In 1882, Heinrich Hertz devoted himself to the study of electromagnetism, including the recent and still generally unappreciated work of Maxwell. Two years later he began his famous series of experiments with electromagnetic waves. During the course of this work, Hertz discovered the photoelectric effect, which has had a profound influence on modern physics.
The effect, as such, is easy to describe: when a substance (typically, a metal) is exposed to electromagnetic radiation it liberates electrons. The number of electrons emitted depends on the intensity of the radiation. The kinetic energy of the electrons emitted depends on the frequency of the radiation. And this is it.
But the explanation is far from trivial. Actually, the explanation of the photoelectric effect was the major work cited in the award to Albert Einstein of the Nobel Prize in Physics in 1921. Einstein’s explanation, proposed in 1905, played a major role in the development of atomic physics. He based his theory on a daring hypothesis, for few of the experimental details were known in 1905. Moreover, the key point of Einstein’s explanation contradicted the classical ideas of the time. He assumed that the energy of light is not distributed evenly over the whole expanding wavefront (as the classical theory assumed). Instead, the light energy is concentrated in separate “lumps.” In addition, the amount of energy in each of these regions is not just any amount, but a definite amount of energy that is proportional to the frequency f of the light wave.
Besides its theoretical and historical importance, the applications of the photoelectric effect are everywhere: from night vision devices to automatic doors or solar panels. And, still, it has a fundamental importance in the study of matter. The analysis of photoemission has become the standard method for the investigation of occupied electronic states, for isolated atoms and molecules and in condensed matter systems, including samples of demanding complexity.
The timing of the photoemission process, i.e., the time elapsing between photon absorption and electron emission, is experimentally accessible since laser sources producing synchronized attosecond extreme-ultraviolet (XUV) pump pulses and few-cycle near-infrared (NIR) waveforms are available and its investigation considerably enhances our understanding of fundamental light-matter interactions. Experiments yield relative delays between photoelectrons from different initial states, which by comparison with appropriate reference states and theory can be turned into absolute values with high precision.
For isolated particles, particularly light atoms, this time interval, which is commonly denoted as (absolute) delay can be calculated with attosecond (10-18 s) precision. Yet, for condensed matter systems, theoretical investigations are much more demanding and mostly limited to one-dimensional model systems or approximations using semiclassical treatments are used. As a matter of fact, suitable high-precision references cannot always be obtained easily and ambiguities may remain.
Now, and in order to resolve these limitations for condensed matter systems, a team of researchers introduces 1 a holistic study of the dynamical photoemission process by rigorously combining time- and energy-resolved attosecond streaking spectroscopy with conventional high-resolution spectroscopy and numerical calculations based on the solution of the time-dependent Schrödinger equation over a broad excitation region spanning from the XUV into the soft-x-ray region.
For prototypical solid materials XUV penetrates deeply, whereas the NIR radiation is reflected or refracted at the surface. Thus the probing of the photoelectrons starts as they traverse the substrate-vacuum boundary and not at their sites of generation. Photoemission delay due to electron transport inside the material dominates at larger kinetic energy, when atomic delays are shown to become small. Inelastic and elastic scattering limit the escape depth to the outermost atomic layers. In general, resonant photoemission, which involves transitions between bulk bands, allows larger escape depth and therefore larger delays than nonresonant photoemission with transitions into waves that do not propagate in the bulk.
No study comparing energy-, angle- and time-resolution and covering resonant and nonresonant photoemission from surface and bulk valence states and from inner-shell states has been performed. This new one covers most of the band structure induced effects, especially those related to the initial state, and elucidates the opposing actions of surface localization, e.g., of a surface state compared to bulk bands, and the extended electron escape depth on resonance.
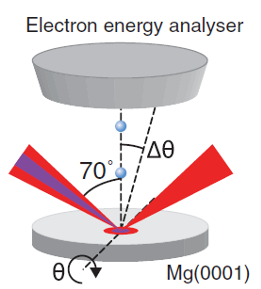
The researchers report measurements of the temporal dynamics of the valence band photoemission from the magnesium (0001) surface and link them to observations of high-resolution synchrotron photoemission and numerical calculations of the time-dependent Schrödinger equation using an effective single-electron model potential. They find that both the observed resonant enhancement and the attosecond retardation of the photoemission are directly linked to the spatial structure of the delocalized sp-band states. By exploiting the synergies between the complementary information obtained from conventional high-resolution photoemission and attosecond streaking they can circumvent the ambiguities that plague relative photoemission time delays without implementation of technically challenging references.
The approach used widens the time-domain window into conventional photoemission establishing a connection between energy-space properties of electronic states in solids and the temporal dynamics of the fundamental electronic excitations underlying the photoelectric effect.
Author: César Tomé López is a science writer and the editor of Mapping Ignorance
Disclaimer: Parts of this article may have been copied verbatim or almost verbatim from the referenced research paper.
References
- Johann Riemensberger, Stefan Neppl, Dionysios Potamianos, Martin Schäffer, Maximilian Schnitzenbaumer, Marcus Ossiander, Christian Schröder, Alexander Guggenmos, Ulf Kleineberg, Dietrich Menzel, Francesco Allegretti, Johannes V. Barth, Reinhard Kienberger, Peter Feulner, Andrei G. Borisov, Pedro M. Echenique, and Andrey K. Kazansky (2019) Attosecond Dynamics of sp-Band Photoexcitation Phys. Rev. Lett. doi: 10.1103/PhysRevLett.123.176801 ↩