How to tear the cell membrane and do not kill it trying: a door to a new in vivo biochemistry.
Robert Hooke discovered the cell in 1665. Plenty of years passed until the human being, in less than three decades, went from elucidate DNA structure (1953) to control artificially the genetic expression of a living cell. Thorough knowledge of the life´s language (genetic code) and information flows (DNA–>RNA–>Protein) was paramount to achieve such a goal. But not only them, once a plasmid-based vector is ready, it has to reach the cell interior, even the nucleus in eukaryotic cells. A wide range of existing techniques aims intracellular delivery of macromolecules as DNA and RNA but also proteins or other materials with relevance in research or clinical therapy. Obviously, all those techniques attempt to permeabilize the cell membrane, which is impermeable to most of those macromolecules. These methodologies range from the chemical modifications, special peptides and liposomes (using endocitical pathways), up to pore generation via electric (electroporation) and sonic (sonoporation) shock. Nowadays, the intracellular delivery of DNA and RNA has high efficiency with almost all of them, but to introduce proteins or other interesting materials remains elusive or at really low efficiency due to shock-induced damage in the material and the cell death. The latest point has been overcome by means of direct microinjection, that despite being effective, it has low throughput and, in addition, requires extremely specialized and expensive equipment.
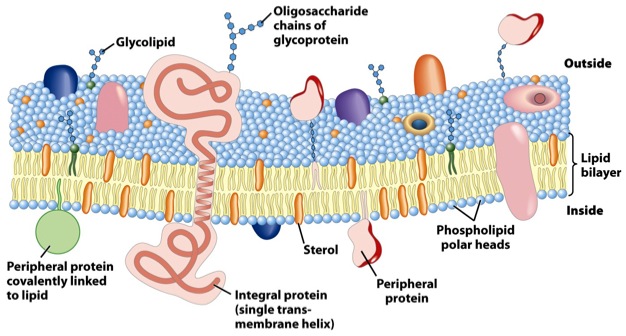
In this paper1, Armon Sharei and a multidisciplinary team describe a device to perform cytosolic delivery of macromolecules by means of mechanical poration. This method is the last implementation, there have been others before 2, of a technique originally developed by Mark F. Clarke and Paul L. McNeil in 1992 3. Using hypodermic needles, they observed transient cell permeablization. It is supposed that shear forces applied to cells increase membrane tension, tearing it and making holes big enough to fit macromolecules. It would be fatal, but cells are provided of mechanisms to repair the membrane, sealing it in about 30-35 seconds after injury, of course, depending on the degree of membrane rupture 4 (Fig.2).
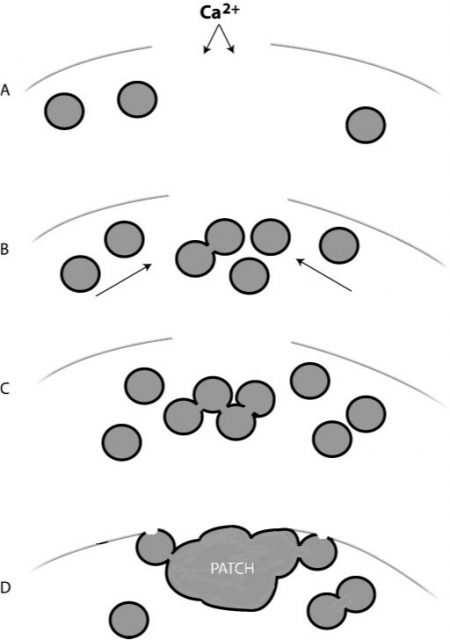
Therefore, the poration is somewhat reversible regardless the method used: indirect (electroporation, sonoporation, etc) or direct (scraping or shared-based). However, in addition to the above-mentioned drawbacks, indirect methods have less control on local conditions, and on the other side, shared-based methods (2, 3) show low viability or efficiency. Sharei´s later development differs from the related ones because it uses a physical constriction to deform and shear the cells (Fig. 3). It is shown as a controlled, reproducible, high cell viability and highly efficient intracellular delivery method.

Their hypothesis is that rapid mechanical deformation of cells results on transient holes formation in the membrane (FIG3). And even more, that the size and frequency of these holes would be a function of the shear and compressive forces affecting the cell on the way through the channel constriction. Therefore, the intracellular delivery of material would occur by diffusion directly from the surrounding media while these holes remain open. To check the hypothesis and optimize the device, labeled 3 and 70-kDa dextran molecules (Polysaccharide) and siRNA were used. Intracellular delivery efficiency of these membrane-impermeable macromolecules was checked in HeLa cells (immortal cell line used in scientific research) modulating different divice´s parameters as cell speed, constriction dimensions, and number of constrictions in the device. They found that the intracellular delivery was directly proportional to those parameters, not due to endocytic processes, consistent with a diffusion-based transport and happening within the first minute after injury induction but not later. Furthermore, a low decrease on cell viability is shown. There after, this technique was applied to several mouse primary cell lines using labeled 3 and 70-kDa dextran molecules as a marker and without device’s cell type optimization. About this, two aspects should be considered. The first is that those cell types are especially difficult to transfect (intracellular delivery of nucleic acids) for most common methodologies. The second is that the two selected dextran sizes range from siRNA similar sizes (3 kDa) to protein similar sizes (70 kDa). All together, pointing out that, under the described conditions, the device is competent to introduce not only nucleic acids but big macromolecules keeping a good level in the cell viability even working with cell types known to be difficult to deal with. Furthermore, antibodies and other not biological sensors and probes like gold nanoparticles, quantum dots and carbon nanotubes were delivered in a living cell cytosol under the same experimental conditions (Figure 4).

Finally, the methodology under review approaches therapeutics, and hence a more applied point of view facing cell reprogramming. There, other endocytosis-related techniques are highly inefficient, due to endosomal trapping of material. An example of cell reprogramming is the transformation of human fibroblast cells in embryonic stem cells able to differentiate in to the three germ layer cell types. This achievement is normally conducted via viral vectors expressing four specific transcription factors (Oct4, Sox2, c-Myc, and Klf-4). The use of viral vectors is almost forbidden in human therapeutics due the risk of pathologies derived from chromosomal integration. To avoid viral vectors, the direct intracellular delivery of purified transcription factors is an interesting option. This new methodology, unlike the endocytosis-based or electroporation ones, performs that task more efficiently. By mean of intracellular delivery of these four specific transcription factors in primary human cells, they obtain, after a month, high amount of colonies expressing embryonic stem cell markers.
Under my point of view, the potential of such a technique is beyond doubt. It is true that its use is quite unlikely regarding nucleic acids intracellular delivery, at least in common cell lines used daily in laboratory. Other easy and cheap methodologies are already working with that aim long ago in scientific research, where the chemical toxicity and/or the risk of vector chromosomal integration are not the big drawbacks. As far as I’m concerned, the greatest advantage of this delivery system (other than the potential in therapeutics) mainly appears in its ability to introduce other large macromolecules into many cells types at high throughput. Proteins or even non-biologic materials could be used as sensors or probes in living cells. This latter point opens a door that could help considerably to understand the molecular mechanisms of life. Traditionally, natural fluorescent proteins have been used as probes in living cell systems used (by cloning their sequences in frame with the protein of interest). They are quite useful but it is really challenging using them to track only few proteins inside the cell due to technical limitations, e.g. it is necessary to reach certain local concentration, usually high, of fluorophore tagged protein to make it visible. Now, we have not only the ability to mark proteins in a test tube with more powerfull non-biological probes, but also to introduce them in living cells using these shear-based delivery systems. The possibility of track unambiguously the intracellular journey and activity of a single marked protein inside a happy living cell in certain moment of its development, but not in other, could answer questions which haunt in biochemists and molecular biologist minds for too long.
References
- Sharei A, Zoldan J, Adamo A, Sim WY, Cho N, Jackson E, Mao S, Schneider S, Han MJ, Lytton-Jean A, Basto PA, Jhunjhunwala S, Lee J, Heller DA, Kang JW, Hartoularos GC, Kim KS, Anderson DG, Langer R, Jensen KF. A vector-free microfluidic platform for intracellular delivery. Proc Natl Acad Sci U S A. 2013 Jan 22 ↩
- Hallow DM, Seeger RA, Kamaev PP, Prado GR, LaPlaca MC, Prausnitz MR. Shear-induced intracellular loading of cells with molecules by controlled microfluidics. Biotechnol Bioeng. 2008 Mar 1;99(4):846-54 ↩
- Clarke MS, McNeil PL. Syringe loading introduces macromolecules into living mammalian cell cytosol. J Cell Sci. 1992 Jul;102 ( Pt 3):533-41 ↩
- McNeil PL, Steinhardt RA. Plasma membrane disruption: repair, prevention, adaptation. Annu Rev Cell Dev Biol. 2003;19:697-731 ↩
1 comment
[…] How to tear the cell membrane and do not kill it trying: a door to a new in vivo biochemistry. […]