Shear-driven drug delivery service
Disruption of normal blood flow to vital organs such as the heart, lung, and brain is the main cause of death in adults in the Western world. Current therapies require hospital facilities, since clot-lysing drugs are administered systemically or through a catheter placed within the obstructed vessel. Besides, the doses are limited by the potential risk of bleeding. To overcome these limitations, Korin et al. 1 propose a drug delivery system based on flow characteristics in narrowed vessels that concentrates the drug in these regions. This simple strategy lowers required drug doses and minimizes side effects while maximizing the efficacy of the treatment.
In normal blood vessels, the shear stress has values below ~70 dyne/cm2 (7 Pa). In case of stenosis or thrombosis, shear stress can increase by one or two orders of magnitude to >1000 dyne/cm2 (100 Pa). When this happens, platelets are naturally activated in these regions, adhering to the surface of the already narrow vessel making it even narrower. Inspired by this natural targeting phenomenon, Korin et al. propose a therapeutic strategy that uses local high shear stress as a generic mechanism to target drug delivery. The shear-activated nanotherapeutics (SA-NTs) are basically aggregates of smaller nanoparticles, and have a total diameter of 1 to 5 m (similar to actual platelets). When blood flows under normal conditions, the aggregates remain intact; but when they are exposed to high local shear stress, they breakup into nanoscale components. Due to the smaller size of the nanoparticles, the drag they experience is smaller, and they can adhere more easily to the walls of the vessel. Adhesion can be further enhanced by coating the particles with molecules that bind to endothelial cells (cells of the inner surface of blood vessels) or other relevant targets.
Experimental tests
The SA-NTs used in the experiments were produced by spray-drying a solution of poly(lactic-co-glycolic acid) (PLGA 50:50, MW 17 kD), obtaining aggregates of () that are composed of small () nanoparticles (Fig. 1A).
Initially, in order to determine the shear-sensitivity of breakup mechanism, Korin et al. applied different controlled shear stresses to SANTs, detecting an 8- to 12-fold increase in the concentration of released NPs when the level of shear reached 100 dyne/cm2 (10Pa) or higher (Fig. 1C).
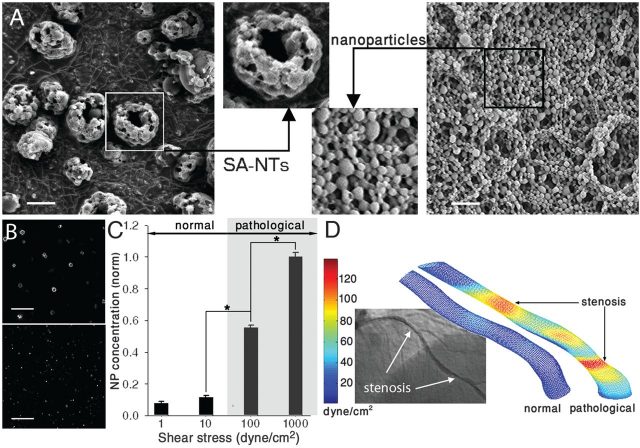
Next, they measured the release of nanoparticles in constrictions under actual blood flow conditions to determine how effective this selective targeting is. Tests were carried out in a three-dimensional microfluidic model of a constricted blood vessel with 90% lumen obstruction made of a silicon-based organic polymer (poly-dimethylsiloxane) (Fig. 2, A and B). CFD simulations of this model revealed that the shear rate at the constriction is increased ~100-fold (Fig. 2C). The experiments showed a 16-fold increase in the release of nanoparticles respect to that of unobstructed channels of similar dimensions (Fig. 2D). Besides, fluorescence imaging confirmed that the nanoparticles accumulated in the narrowed region, while very few were released upstream of the constriction (Fig. 2E).
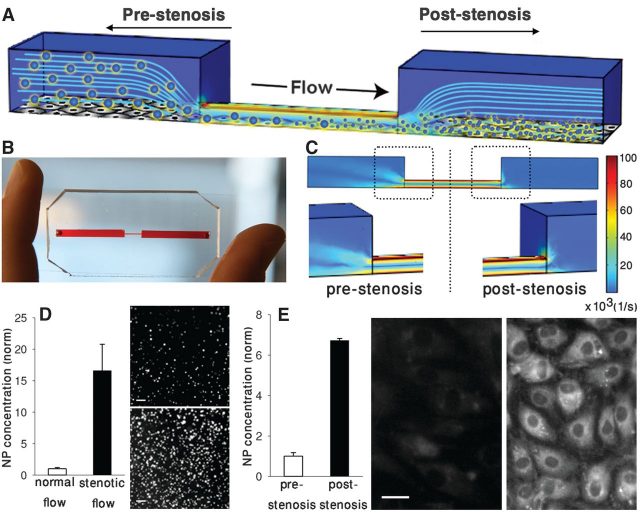
The ability of SA-NTs to dissolve blood clots was tested in vivo using fluorescent tPA-coated nanoparticles in mice. tPA (tissue plasminogen activator) is a thrombolytic agent which has high affinity for a protein involved in the clotting of blood, called fibrin. In this case, they triggered clot formation by injuring the vessel wall by direct exposure to ferric chloride. This lead to the appearance of large blood clots within minutes in injured mesenteric arteries (~100 m diameter, normal wall shear stress ~30 dyne/cm2) that decreased the effective diameter by more than 80% (Fig. 3, B and C) and caused the local shear stress to rise to ~450 dyne/cm2. The fluorescent nanoparticles allowed, on the one hand, the visualization of these lesions (Fig. 3B). On the other hand, they progressively eroded the thrombi, with complete elimination of the obstruction 5 min after SA-NT injection (Fig. 3, B and C). Unobstructed vessels were also monitored during 15 minutes, revealing that the SA-NTs circulated through them without disruption into nanoparticles. In this experiment, tPA nanoparticles from SA-NTs were able to reopen the injured vessel and delay the time to its occlusion for a longer time than PBS (phosphate-buffered saline), and predissociated tPA nanoparticles (Fig. 3, C and D).
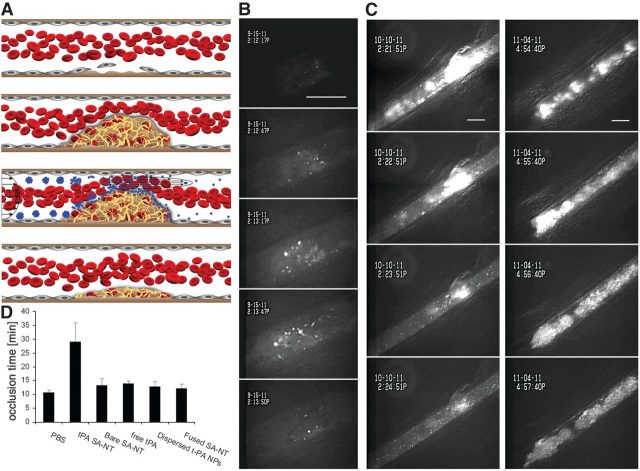
Next, they tested SA-NTs for treatment of experimentally induced fibrin clots in vitro, to determine their potential with life-threatening embolic occlusions. Fibrin clots were injected into microfluidic channels with constrictions (80 m high, 500 m wide), and lodged in there partially obstructing the flow (Fig. 4A). An infusion of SA-NTs (100 mg/ml) with tPA (50 ng/ml) at physiological flow rates was able to reduce the clots by one half within an hour of treatment (Fig. 4A). The same treatment with predissociated tPA had less than 5% reduction in clot size.
The same fibrin clots were infused in an ex vivo whole-mouse-lung ventilation-perfusion model. Multiple microemboli appeared (Fig. 4C), causing the pulmonary artery pressure to increase from 8 to 30 mm Hg (Fig. 4E). Then, they perfused the same tPA-coated SA-NTs, that is 100-mg/ml of aggregates containing 50 ng/ml tPA, at physiological flow rate. Experiments showed a >25-fold increase in accumulation of NPs at the occlusions, (Figs. 4, C and D). Within one hour of treatment, the pressure levels of the pulmonary artery went back to normal. The same treatment with predissociated tPA -and even 10 times more- had no significant response (Fig. 4F). To get similar effects to SA-NTs, a 100-times-higher concentration of free tPA had to be administered.
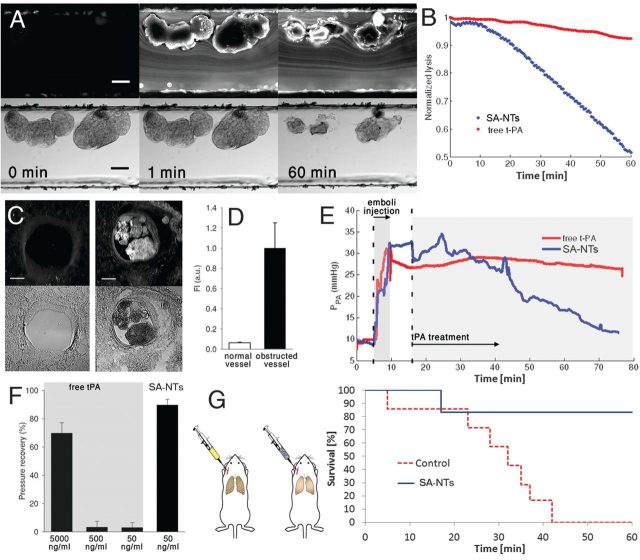
The following step was to test SA-NTs in living mice with pulmonary embolism. Fluorescent fibrin clots were infused into the jugular vein of mice, and accumulated in peripheral blood vessels in the lungs. Then, tPa-coated SA-NTs were administered either immediately after clot injection, or 30 minutes later. The experiment showed that tPA-coated SA-NTs reduced by >60% total clot area and clot number when administered immediately after clot infusion, and by >30% when infused after half hour.
Finally, SA-NTs were tested in mice for treatment of life threatening acute massive embolism. They infused a solution of large fibrin clots ( diameter; ~ 100/injection), which accumulated in the main pulmonary arteries. The mice were immediately infused with tPA-coated SA-NTs, or with carrier fluid as a control group, during 45 min. All animals from the control group died within 1 hour after infusion of the clots (Fig. 4G), while 6 out of 7 of the treated mice survived. Moreover, none of the SA-NTs– treated animals displayed any symptoms of respiratory distress after the experiment.
Conclusions
Korin et al. have proved through exhaustive experiments that SA-NTs provide safer thrombolytic therapies with smaller doses without compromising the effectiveness of the treatment. Additionally, thanks to their fluorescent coating, they were proved useful to visually detect occlusions in vessels.
Even though the findings reported in this paper are very promising, the authors are cautious and talk about extensive clinical studies that will be necessary to fully prove this approach. To an outsider to medical research as myself, all these experiments may seem redundant, and is surprising to find out that there are actually hundreds of rules and regulations about all the tests required before being able to use a medical treatment in humans.
References
- Netanel Korin, Mathumai Kanapathipillai, Benjamin D. Matthews, Marilena Crescente, Alexander Brill, Tadanori Mammoto, Kaustabh Ghosh, Samuel Jurek, Sidi A. Bencherif, Deen Bhatta, Ahmet U. Coskun, Charles L. Feldman, Denisa D. Wagner, and Donald E. Ingber. Shear-Activated Nanotherapeutics for Drug Targeting to Obstructed Blood Vessels. Science, 10 August 2012: 337 (6095), 738-742. ↩